Fig. 4.1
Somatic and stem cell stress response mechanisms are shared for acute and chronic and low, moderate, and high stress levels for cellular homeostasis. These responses tend to be cell autonomous. But stem cells have a unique capability of stress induced differentiation that is part of an organismal response to provide essential function during acute and chronic moderate-high stress exposures that diminish stem cell population expansion. In the first part of the chapter, mechanisms of the stem cell cellular homeostasis response will be analyzed and in the second part, mechanisms of the organismal homeostasis response through differentiation will be analyzed
4.2 Early events in Embryogenesis; Balancing Anabolism and Stress During Early Embryonic Programming
The embryo in vivo and in culture faces daunting anabolic and programmatic requirements. Rapid stem cell proliferation occurs at a unique period when all or most cells are dividing stem cells and morphogenetic events such as cavitation in the preimplantation embryo require high amounts of energy. In both humans and mice, sufficient anti-luteolytic placental hormones must be developed from the first placental lineage within days after implantation when no mRNA for the relevant hormones is detected in blastocysts before implantation. A very small embryo must expand stem cell populations rapidly after implantation and differentiate a subpopulation of cells to sustain ovarian progesterone production or the embryo is lost (Xie et al. 2011). This is difficult normally but when stress has diminished stem cell accumulation it is made even more difficult. The embryo requires energy to mediate transcription of approximately 4,000 genes at the 2-cell stage and another 5,000 genes at the 8–16 cell stage (Hamatani et al. 2004; Wang et al. 2004). Anabolic activity, requiring a high energy input is substantial during formation of the first epithelium and blastocoel cavity (Donnay and Leese 1999; Brison and Leese 1994). In these early cell divisions, the embryo begins the necessary complete reorganization of the use of its genome during the transition from maternal to embryonic control. The embryonic program develops many unique aspects compared to the programs of the gametes that formed the embryo, but stress slows program development and cell proliferation (Xie et al. 2011). The embryonic programs prepare the embryos for the events before and after implantation (Armant 2005).
4.2.1 Defining Stress and Categorizing Classes of Stress by the Transcription Factors in the Survival Responses of Somatic Cells, Stem Cells and Embryos
In 2007, the U.S. National Research Council at the National Academy of Sciences produced an overview of the kinds of stress and stress mechanisms that somatic cells respond to (National Research Council (U.S.) 2007). This overview can be further defined in terms of response elements of transcription factors (TFs) activated by each type of stress (Simmons et al. 2009). The stress-TF pairs analyzed were; (1) oxidative stress-NRF2 (nuclear factor elytroid-derived factor 2), (2) heat shock response-HSF1 (heat shock factor-1/Table 4.1), (3) DNA damage-p53/TRP53 (Table 4.1), (4) hypoxia-HIF1 (hypoxia inducible factor-1; Table 4.2), (5) ER stress-XBP1/ATF4/ATF6 (X-box binding protein 1/activating transcription factor 4/6), (6) metal stress-MTF1 (metal regulatory transcription factor 1), (7) Inflammatory stress-NFKB (nuclear factor kappa-light-chain-enhancer of activated B cell), and (8) hypertonic stress-NFAT5 (Nuclear factor of activated T-cells 5). Some of these TFs are not specific to single stress signaling pathways and can be activated by multiple pathways; p53 is activated by culture stress of embryos (Jin et al. 2009) as well as genotoxic stress, ATF4 is activated by hypertonic stress of TSCs (Liu et al. 2009), as well as ER stress. Other stress-induced TFs like the FOXO family also play important roles in the embryonic stress response (Xie et al. 2011). The regulation of the TFs by stress enzymes was not analyzed in detail in this review (Simmons et al. 2009). However, SAPK/JNK and p38 -mitogen-activated protein kinase (p38MAPK), as well as protein kinase (PK)A and phosphotidyl inositol 3 kinase (PI3K) play important roles in these canonical, and thus well characterized, stress pathways. But, multimerized response elements bound on the promoter by these transcriptions factors linked to luciferase reporters allow high throughput screening of stress stimuli in somatic and stem cells (Chandler et al. 2011) that should hasten our understanding of the kinetics, magnitude, and interactions of different types of stress.
Table 4.1
Stress enzyme and other stress mediators are important in embryonic and placental development, as illustrated by null mutants that have increased lethality during stressed pregnancies or embryo or stem cell culturea
Stress gene | Site of placental or decidual expression or ubiquitous expression | Period when stress leads to lethality or deviation from normal development | Reference |
---|---|---|---|
TRP53 | Ubiquitous expression (MDM2) | E3.5. Culture stress diminishes progressing To blastocyst or successfully reimplanting On some genetic Backgrounds | |
HSF2 | Ubiquitous expression | E3.5 cell survival in stressed blastocyst | (Le Masson and Christians 2011) |
MEKK4 | Ubiquitous expression | E7.5. MEKK4 null TSC can be isolated but die during passage in culture due to EMTb blocked by MEKK4 and SAPK | (Abell et al. 2009) |
Oct1 | Trophoblast lineage | E7.5-9 Necessary to isolate TSC at E3.5, but in vivo some null sustain some TSC proliferation through E7.5 | (Sebastiano et al. 2010) |
PLPA * | Villous trophoblasts | Insufficient response to Peak expression E10.5 Hypobaric O2 and failure of normally Trophoblast to NK maternal Response and villous response E9.5 | (Ain et al. 2004) |
Table 4.2
Factors resulting in increased or decreased number of cells.
CoQ10, improved mitochondrial function | Increased TE + ICM cell number in cultured embryos | (Stojkovic et al. 1999) |
Culture at 2 % more than 0.5 % oxygen levels | Increased proliferation rates in muTSCs | (Zhou et al. 2011) |
Culture at 2 % more than 20 % oxygen levels | Increased proliferation rates in hu/muTSCs | |
Culture at 5 % more than 20 % oxygen levels | Increased cell number in cultured embryos | (Wale and Gardner 2013) |
Culture in least more than most stressful media | Increased cell number in cultured embryos | (Xie et al. 2006a) |
FGF4 in embryo culture | Increased TE and Endoderm cell number | |
FGF4 in embryo culture | Increased TE cell number/decreased differentiation | (Rassoulzadegan et al. 2000) |
GMCSF in embryo culture; Stem cell survival | Increased cell number in cultured embryos | (Robertson et al. 2001) |
Improved media | Increased cell number in cultured embryos | (Erbach et al. 1994) |
Insulin/IGF in embryo culture | Increased cell number in cultured embryos | (Rappolee et al. 1992) |
Leptin in embryo culture | Increased cell number in TE > ICM | (Kawamura et al. 2003) |
PAF in embryo culture | Increased cell number in cultured embryos | |
P38MAPK/MAPK activation | Increased cell number in cultured embryos, inhibitors decrease cell number | (Madan et al. 2005) |
Progranulin in embryo culture | Increased cell number in TE > ICM | (Qin et al. 2005) |
TGFα or higher density embryo Culture, stem cell survival | Increased cell number in cultured embryos | (Brison and Schultz 1997) |
Decreased matrix stiffness | Increased cell number in cultured embryos | |
Microfluidics culture, embryos | Increased cell number in cultured embryos | (Heo et al. 2010) |
Pulsatile micro-vibration | Increased cell number during embryo culture | (Hur et al. 2013) |
Embryo handling without culture | Epigenetic effects | (Rivera et al. 2008) |
Pipetting shear stress | No affect on cell number if short exposure | (Xie et al. 2007a) |
Adrenaline in embryo culture | Decreased mouse preimplantation growth, ICM > TE | |
Cortisol in stem cell culture | Decreased human placental cell growth | (Mandl et al. 2006) |
Hyperosmotic stress | Decreased cell number during embryo culture | |
Genotoxic stress | Decreased cell number during embryo or TSC culture | |
Maternal Malnutrition in vivo during preimplantation development | Decreased cell number TE and ICM | (Kwong et al. 2000) |
SAPK activation | Decreased cell number during embryo or TSC culture | |
Shear stress | Decreased cell number during embryo culture | (Xie et al. 2006b) |
Stress to induce stress tolerance | Decreased cell number during embryo culture | |
TRP53 activation in embryo culture | Decreased cell number during embryo culture | (Chandrakanthan et al. 2006) |
4.2.2 Integrating Maternal Nutritional/energy Status to Enable Peri-Implantation Embryogenesis to use Energy for Normal or Stressed Development
Maternal nutritional status determines readiness for early embryonic development and implantation as well as the correct induction of the programs of the conceptus.
Leptin is an endocrine and paracrine hormone that signals sufficiency of maternal fatty acid reserves and is a well-known regulator of maternal sexual maturity and continuing readiness for pregnancy during the fertile period of mouse and humans [Chap. 47, (Knobil and Neill 2006)]. In humans, maternal leptin is upregulated after feeding and negatively feeds back on appetite but also controls reproductive homeostasis through endocrine regulation of initiation of menarche and enabling continuing menstrual cycles.
Global endocrine regulation is through secretion by adipocytes and a small set of other cell types, but leptin is also secreted by the oviductal and uterine epithelium and is mitogenic for the embryo in culture and regulates trophoblast invasiveness (Xie et al. 2011; Kawamura et al. 2003; Gonzalez et al. 2000a, 2000b). This is an example of the integration of the global and local maternal signaling that enhances embryonic development when the maternal nutritional status can support the preparation for implantation.
However stress diverts energy away from essential, normal, high energy requirements and leads to slowing of preimplantation embryogenesis, stem cell proliferation, and morphogenesis (Xie et al. 2011). We define stress as any external stimulus to the embryo that negatively affects normal trajectories of development and growth. Stress induces changes in cell metabolism, cell cycle progression, slowed protein synthesis, vesicular trafficking, phosphorylative regulation of enzymatic activities, alterations in cytoskeleton (Xie et al. 2011; Mansouri et al. 2012), and slower changes in the transcriptional program to mediate long-term adaptation (de Nadal et al. 2011). The immediate need of stem cells in the conceptus is to survive, but longer term adaptation requires that sufficient stem cell subpopulations differentiate to provide sufficient function.
Accumulating data from animal and human studies indicate that prolonged exposures of the developing embryo during peri-implantation development to various stimuli/stress such as physiological or emotional stress or deficient nutrition can have profound implications for early embryonic and fetal development, and for postnatal life. Maternal stress hormones such as adrenaline and cortisol have the capacity to slow growth of the preimplantation embryo and its stem cells (Xie et al. 2011). After implantation the placenta is important in modulating maternal stress due to maternal psychological distress, and the potential mechanism through which maternal psychological stress shapes development is through epigenetic changes which regulate gene activity (Monk et al. 2012). Studies in humans demonstrate that high circulating levels of maternal cortisol during pregnancy correlate negatively with birthweight, suggesting that excess glucocorticoids can cross the placental barrier. It is thought that if the placental enzyme, 11β-hydroxysteroid dehydrogenase type (HSD)2, which converts active glucocorticoids into inactive products, is deficient or is overwhelmed by high maternal cortisol production, the fetus grows more slowly. The diminished HSD2 function may be due in part to alterations in DNA methylation at CpG loci within HSD2, and in methylation of glucocorticoid receptor genes important in regulating cortisol levels, tissue glucocorticoid action, blood pressure and fetal growth (Drake et al. 2012; Burton and Waddell 1999). Deficiencies in HSD2 enzyme, potentially increase fetal glucocorticoid exposure, but can also arise in association with maternal stress, malnutrition and diseases such as hypertension (Reynolds 2013). Severe maternal nutritional deficiency and psychosocial stress, correlated with higher cortisol during the first and/or second trimesters of pregnancy, leads to shorter gestation delivery of small for gestation age (SGA) (Entringer et al. 2011; Seckl and Holmes 2007) babies and abnormal post-natal development. Prenatal maternal anxiety in the third trimester is associated with increased epigenetic modification of the glucocorticoid receptors (Oberlander et al. 2008) that is correlated with increased postnatal salivary cortisol response.
These stressors may have a direct effect on the embryo and act by dysregulating the hormonal environment, or via epigenetic modification of genes important in early life events that lead to increased, subsequent disease risk. Abnormal post-natal developmental conditions include antisocial personality disorder (Neugebauer et al. 1999), mood disorders (Kleinhaus et al. 2013), hypertension, and diabetes (Kaijser et al. 2009). Maternal psychological stress during pregnancy may also exert a “programming” effect on the developing telomeric system that is apparent at birth, as reflected in newborn leukocyte telomere length (Entringer et al. 2013), which in adults has been shown to be a predictor of age-related diseases and mortality (Entringer et al. 2012). It has been shown that telomere length is maintained in healthy cells early during life but that telomeres shorten with age or after stimuli that induce premature aging. In experiments in sheep, undernutrition during the period of superovulation and early embryonic development reduces the total number of viable embryos collected (Abecia et al. 2013). Thus, maternal hormones and nutritional and stress status can regulate the growth rate of the early embryo negatively or positively.
During the preimplantation period embryonic and trophoblast stem cells (ESCs and TSCs, respectively) arise and endoderm is segregated from the ESCs of the inner cell mass (ICM) of the blastocyst. Signaling trophoblast giant cells (TGCs) are derived from the TSCs as the blastocyst implants, integrate and signals for maternal support and prepares for gastrulation (Fig. 4.2). One function of TGCs and endoderm at implantation in mouse is to induce maternal secretion of nutrients and the absorption and transcytosis of these nutrients, respectively (Xie et al. 2011; Rappolee 1999). From TSCs, all rodent TGC produce hormonal signals, and placental lactogen (PL)1 is the first, detectable placental hormone in maternal blood—within 36 h of implantation (Fig. 4.2) and this hormone induces progesterone secretion in the corpus luteum. Progesterone induces high glycogen secretion by maternal tissue near the surface of the placenta occurring especially early in the first trimester (Shapiro et al. 1980; Salameh et al. 2006; Burton et al. 2002). From ESCs, the primitive and then subsequent visceral endoderm of the implanting embryo has produced megalin/LRP2 cubilin, and DABs (Gerbe et al. 2008; Frankenberg et al. 2011), the lipid-acquiring complex that is necessary for HNF4-dependent maintenance of adjacent embryonic ectoderm by E6.0 (Rappolee 1999). Thus the first, essential differentiated lineages from ESCs and TSCs at implantation have a common function of nutrient acquisition to sustain rapid stem cell proliferation.
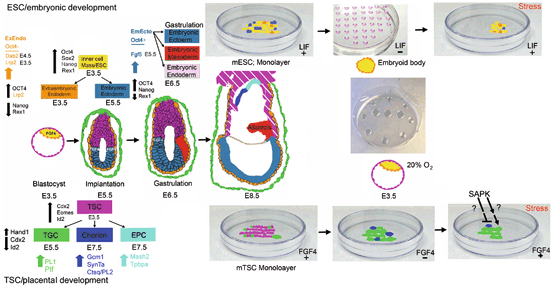
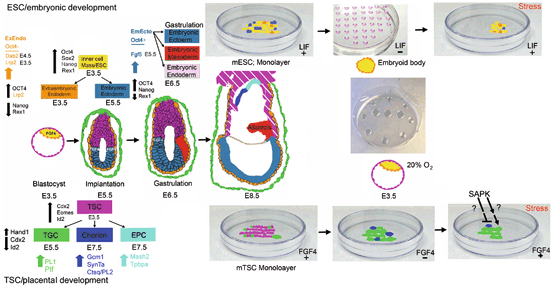
Fig. 4.2
Peri-implantation development involves the initial determination of placental and embryonic stem cell lineages, the further differentiation of extraembryonic endoderm from ESC lineage and TGC from TSCs. The central four diagrams in sequence from Preimplantation E3.5 blastocyst to E8.5 late gastrulation show the positions of color-coded ESC/embryonic and TSC/placental development with markers at top left and bottom left, respectively. At top right and bottom right are shown the maintenance culture of ESC with LIF and TSC with FGF4, respectively. Note that multiple colors of cells during maintenance culture indicates the heterogeneity with respect to stemness and subpopulations spontaneously allocating or differentiating the first lineage. To the right of maintenance culture is differentiation culture with hanging drop embryoid bodies of ESC without LIF or TSC monolayer without FGF4. Center right is the typical microdrop under oil culture of preimplantation embryos or blastocyst outgrowths to emulate peri-implantation development of whole embryos. Note that stress can be dominant to LIF for ESCs or FGF4 for TSCs and induce differentiation to first lineages despite the presence of these two potency-maintaining growth factors
Regulation of energy metabolism within the oocyte and embryo before implantation is also very important for providing for key morphogenetic and molecular events. The oocyte and embryo have energy stores, largely in the form of triglycerides, but consume exogenous nutrients throughout preimplantation development . For example, in the mouse, there is an obligatory requirement for pyruvate in the zygote and first cleavage division embryo followed by a switch to glucose at compaction at the 8-cell stage (Brison and Leese 1991). Mitochondrial function at the 2-cell stage in converting pyruvate to ATP is under partial regulation by NACHT, leucine-rich repeat and PYD-containing 5 (NALP5)/Mater (Fernandes et al. 2012) (Table 4.3) which is a null lethal at this stage. Decreased efficiency of ATP production by mitochondria, possibly exacerbated by abnormal cytolocalization, leads to increased activity and ROS-mediated damage in the Mater null mutant. Increased mitochondrial activity and its proper distribution throughout cells in the embryo requires caudal-related homeobox protein (Cdx)2 transcription factor (TF) by the 8-cell stage (Wu et al. 2010). Although mitochondrial activity is present through oogenesis, cleavage divisions and blastocyst development (Leese 2012), it is likely to be cytolocalized in different cells in different ways during development (Batten et al. 1987) and to require upregulation, and downregulation of electron transport chain (ETC) activity (Xie et al. 2014; Van Blerkom et al. 2006) at different stages during preimplantation development. This downregulation of the ETC will be discussed in more detail in Sect. 4.2.3.
Table 4.3
Stress enzyme and other stress mediators are important in embryonic and placental development, as illustrated by null mutant lethal phenotypesa
Stress gene | Onset, site of placental expression earliest noted deviationb | Onsetb, cause of lethality | Reference |
---|---|---|---|
NLRP5/Mater | 2-cell embryo | E1.5 Mitochondrial localization is Abnormal and ATP synthesis is Inefficient. High ROS created due to overactive compensation of Mitochondrial function. Arrest at 2-cell stage | Fernandes et al. 2012 |
Ppp1r15a/b | Preimplantation ubiquitous (eIF2α)/ER stress | E3.5 Failure to dephosphorylate eIF2α after normal stress activates it | Harding et al. 2009 |
Glutamine | Preimplantation ubiquitous | E3.5 Embryonic inability to detoxify ammonium at blastocyst stage in vivo Exacerbated by culture at 20 % O2 > 5 % O2 | He et al. 2007 |
JunB | Ubiquitous expression | E10.0 Failure to vascularize villous core of labyrinthine placenta | Schorpp-Kistner et al. 1999 |
HuR | Unique cytoplasmic in ER stress Villous epithelium Deviated at E8.5 c | E10.5 Failure to initiate labyrinthe development; required in epithelium, villous invasion, vascularization and villous branching | Katsanou et al. 2009 |
p38MAPKα | Significant and required Cellular stress, in villous epithelium Deviated at E10.5 | E10.5 Failure of villous enzyme) Vascularizationb after invasion | Mudgett et al. 2000 |
HIF1β/ARNT | Significant and required (Hypoxic stress) in villous epithelium Deviated at E8.5 c | E10.5 Failure of villous vascularization after invasion | Adelman et al. 2000 |
HSP90β | Ubiquitous Heat stress deviated at E10 | E10.5 Failure of villous Vascularization, yolk sac vascular failure E9.5-10.5 | (Voss et al. 2000) |
IRE1 | In villous epithelium In villous epithelium In villous epithelium E13.5 (only test time) | E13.5 Failure of villous vascularization after invasion | Iwawaki et al. 2009 |
Mfn2 | Ubiquitous (smaller embryos at E8.5 c | E11.5 Failure to make energy, TGC layer | Chen et al. 2003 |
There is a major role for the regulatory protein AMP-activated protein kinase (AMPK) which is as an energy sensor. Low ATP Levels reflected by an increase in AMP:ATP ratio activates AMPK, which, once activated, switches off ATP-consuming pathways and switches on catabolic pathways to increase ATP (Hardie 2003; Carling 2004). Besides regulating energy status, AMPK plays a potential role in mediating stress-induced loss of potency and differentiation , TSCs and ESCs (Xie et al. 2013a) as well as regulating mitochondrial function (Wu et al. 2010). Although AMPK can be activated by other pathways and is not always exclusively a biomarker for AMP increase and ATP decrease, it is likely that elevated AMPK activity in stressed 2-cell stage embryos (Xie et al. 2013a) indicates the depletion of ATP.
Many stressors activate AMPK which mediates loss of Id2 protein and phosphorylated Cdx2 protein, the form of Cdx2 which blocks differentiation in intestinal stem cells and may also block TSC differentiation in the early embryo (Xie et al. 2013a). AMPK is a heterotrimeric complex with a catalytic α subunit and two regulatory subunits (β and ϒ) (Stein et al. 2000). The N-terminus of the α-subunit contains a serine/threonine protein kinase catalytic domain and the C-terminus interacts with the other subunits. Activation of AMPK requires phosphorylation at Thr172 on the α subunit. Molecular identification of the upstream kinases is difficult, although liver kinase (LK)B1 and calmodulin kinase kinase (CaMKK) are the two main, physiologically relevant candidates for activating mammalian AMPK (Woods et al. 2003). Cdx2 knockdowns and knockouts lead to lethality at the blastocyst stage (Wu et al. 2010; Strumpf et al. 2005; Chawengsaksophak et al. 1997), but maternal Cdx2 may be important in initiating mitochondrial membrane charge and energy production before the blastocyst stage. Cdx2 may be important (with Oct4 and Id2) in maintaining the potency of totipotent blastomeres before ESC and TSC lineage allocation. However, the Cdx2 phosphorylated at serine60 that is depleted by stress may decrease potency but not induce new transcription as this also requires upregulation of differentiation factors. Stress effects and integration of cellular ATP and differentiation by AMPK will be discussed Sect. 4.3.1.
The kinetics of stress-induced AMPK activity is similar in blastocysts and the TSCs and ESCs derived from them (Zhong et al. 2010), suggesting similar mechanisms of activation. AMPK mediates suppression of anabolism of TSCs (Zhong et al. 2010) and AMPK plays a role in the proliferation and differentiation of ESCs (Wu et al. 2010; Strumpf et al. 2005; Chawengsaksophak et al. 1997). AMPK activation inhibits ESC proliferation by inducing G1/S arrest and promotes the differentiation of ESCs by loss of potency and predisposing towards a few differentiated lineages (Dzeja et al. 2011; Chae et al. 2012). Thus AMPK integrates stress-induced decreases in anabolism and possibly mitochondrial function, and associated reduction in growth rate in both TSCs and ESCs.
Energy reserves from the oocyte and ongoing access to nutrients in the maternal environment also enable robust stress responses in embryos and their stem cells (Xie et al. 2011). In somatic cells, serum starvation (i.e. analogous to malnutrition in vitro) leads to an increase in magnitude and rapidity of stress-induced AMPK activation in response to depleted ATP during hypoxic stress (Liu et al. 2006). This also leads to suppressed proliferation through tuberous sclerosis complex (TSC)2 in somatic cells. This mechanism has not been studied in early embryos or their stem cells.
An interesting model is adolescent pregnancy in sheep, where the still-growing dam competes for nutrition with the growing conceptus in response to over-nutrition which increases maternal mass at the expense of the conceptus (Wallace et al. 2001). In response to gestational over-nutrition, placental development and low progesterone early in pregnancy are associated with low birthweight, and early progesterone supplementation increases birthweight without increasing placental cotyledon number (Wallace et al. 2003). It was concluded that early progesterone replacement may increase placental vascularity or directly affect the inner cell mass growth rate. Reduced fetal cotyledon number was associated with over-nutrition only during the first trimester in sheep, and switch to a moderate diet did not affect cotyledons although it did increase neonatal placental mass and birthweight (Wallace et al. 1999). In the rodent, maternal malnutrition during preimplantation embryogenesis in vivo leads to decreased cell number in both embryonic stem cells of the inner cell mass and the outer trophoblast stem cell lineages at the blastocyst stage (Kwong et al. 2004, 2000). In the second part of the chapter we develop the hypothesis that diminished stem cell accumulation marks the threshold where metabolic stem cell survival mechanisms divert sufficient energy from anabolic growth and trigger the differentiation necessary to develop sufficient differentiated function from fewer cells.
4.2.3 Stress During IVF/ART
Manipulation of embryos in vitro causes elevated stress . Examples include hyaluronidase treatment and handling in ambient bicarbonate buffers such as M2 which activates AMPK (Mansouri et al. 2012) and endoplasmic reticulum (ER) stress (Abraham et al. 2011). Culture media have improved over the past 20 years, but even the most recent, which claim to minimize stress, activate significantly more stress-activated protein kinase (SAPK, Figs. 4.3, 4.4, 4.5, 4.6, 4.7) than in time-matched embryos ex vivo. Moreover, embryos cultured for 4 days have more SAPK activity than those cultured for 2 days (Wang et al. 2005), suggesting that culture stress is ongoing and requires a continuing energy drain. Although this could be a developmental stage-specific effect for embryos, two lines of evidence argue against this. One is that increases in enzyme activities over time in the embryo are not nearly as great as the difference between levels in embryos ex vivo compared with those grown in vitro. The second line of evidence is derived from cultured TSCs maintained with FGF4 and subject to very low hyperosmolar stress (10 mM or 25 mM sorbitol), and thus not progressing rapidly into a different stage, when SAPK increases with time (Zhong et al. 2007). More stressful media also create greater epigenetic deviation leading to the induction of a mechanism which gives rise to long-term negative effects (Doherty et al. 2000) and many postnatal effects (Gosden et al. 2003; Thompson et al. 2002). Pipetting causes stress (Xie et al. 2007a) as does culture at 20 % oxygen compared to 5 % oxygen (Rinaudo et al. 2006). Even embryo transfer without culture leads to epigenetic changes (Rivera et al. 2008). Recently, development of microfluidics devices, in which there is avoidance of shear stress along with optimization of flow, enhances the stem cell population in cultured preimplantation embryos to reach ~ 80 % of time-matched embryos ex vivo (Heo et al. 2010). Stress due to culture induces increased glucose uptake, respiration and lactate production within 1–6 h of culture of post-compaction embryos ex vivo (Gardner 1998; Leese 2002). Thus manipulation of embryos during IVF/ART has been shown to induce stress responses or epigenetic changes linked to stress responses.
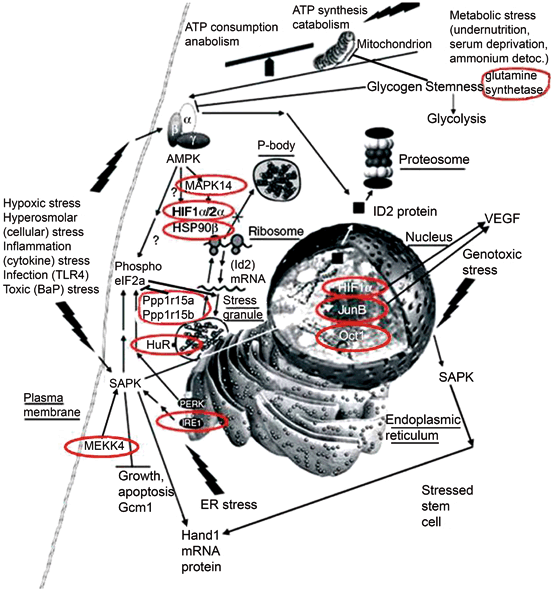
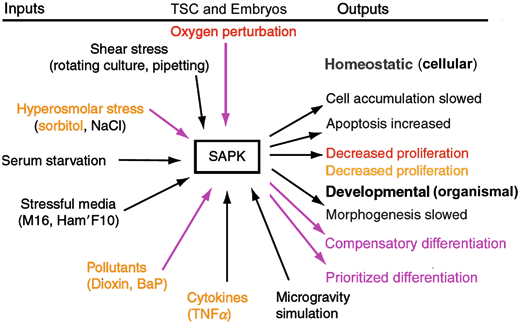
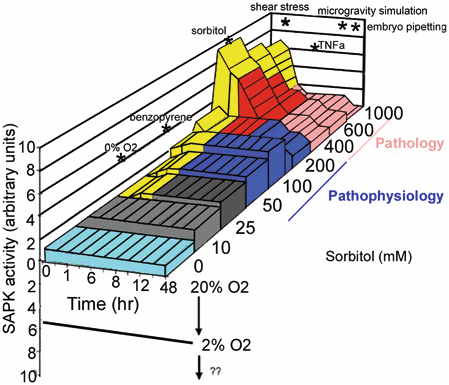
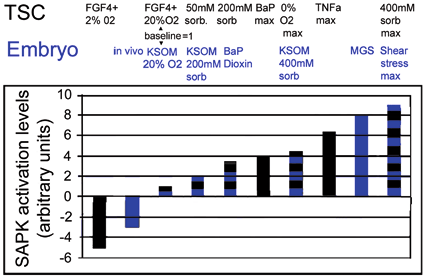
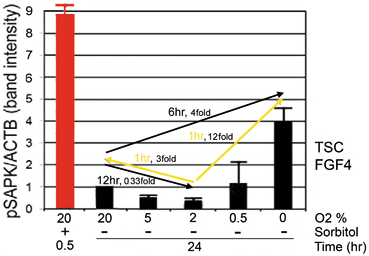
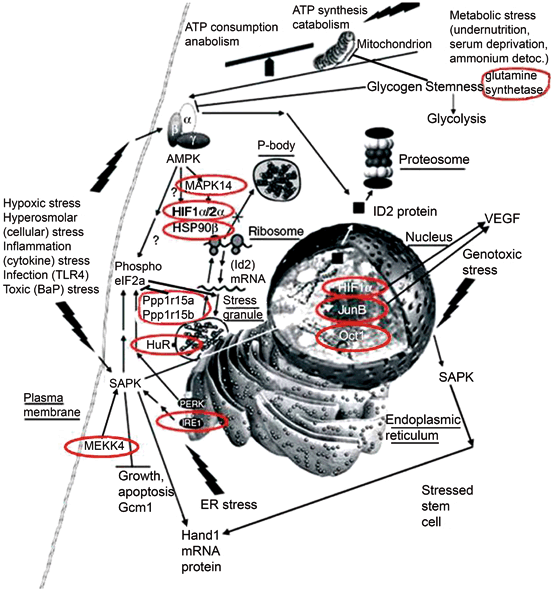
Fig. 4.3
Essential stress pathways in embryos for TSC lineages initiate at the stem cell surface, cytosol, organelles, and nucleus as defined by analysis of lethal null mutations. Red circles show genes from lethal null mutants that mediate homeostatic and developmental outcomes that are part of the adaptive stress response to multiple peri-implantation stressors. One common signaling intermediate from all cell locations is the ATP/AMP ratio for AMPK. SAPK is activated by many pathways originating at plasmalemmal focal adhesions, and signaling receptors such as TLR4 and TNFa, IRE1 in the endoplasmic reticulum or ATM activated by DNA damage in the nucleus. (Modified from Rappolee et al. 2013)
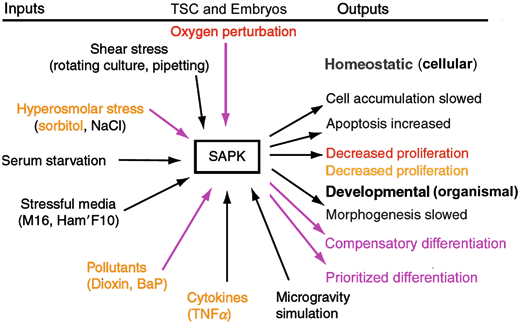
Fig. 4.4
Stress inputs and outputs mediated by SAPK in peri-implantation embryos and TSCs. Purple are stress inputs that induced outputs of compensatory and prioritized differentiation. In Orange are stress inputs that generate an S-shaped dose response for pSAPK and a reverse S-shaped curve for TSC proliferation. Red is a stress input that generates a U-shaped curve for pSAPK and an inverted U-shaped curve for TSC proliferation. For the U-shaped curve, the lower pSAPK activity increase dose is on left side and due to contradictory signals of FGF4 and incorrect O2. The higher pSAPK on the right, hypoxic O2 < 2 % cause highest effects of differentiation and at very severe hypoxia, apoptosis. See Fig. 4.6. In either S or U shaped curve SAPK activity is inversely correlated with stem cell proliferation and embryo developmental rates. (Modified from Xie et al. 2011)
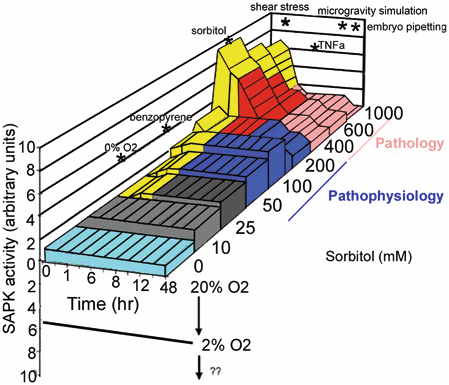
Fig. 4.5
A Stress contour map relates time- and dose-dependent SAPK activity in embryos and TSCs with biological outcomes. A Stress contour map for levels of activated SAPK in peri-implantation embryos and TSCs stimulated by hyperosmolar sorbitol. Yellow shows pSAPK levels during early peak activation dependent on [sorbitol]. Sorbitol dose range and pathologic and pathophysiologic outcome ranges are shown on the right. Blue shows levels of sorbitol that create a “runting model” or slowed growth and increased compensatory and prioritized differentiation. This pathophysiological is the best low-moderate dose that induces most robust loss of stem cell growth with sufficient energy remaining to induce compensatory and prioritized differentiation . Red and Pink show timing and sorbitol doses that induce apoptosis after early peak pSAPK. Pathological doses of stress are so high that compensatory and prioritized differentiation may be initiated (as at 400 mM sorbitol) but most cells die, or if the dose is very high (1000 mM) SAPK is not activated and Hand 1 is lost. On the “walls” of the 3D graph are the maximal pSAPK for other types of stress. At the bottom of the figure, it should be noted that almost all stressors here and in Fig. 4.2 were tested during culture at 20 % O2 where SAPK was set to the baseline “1”. More recent studies (Zhou et al. 2011) show that 20 % O2 induces stress in TSCs as a contradictory signal to FGF4 and this reduces pSAPK 3–5fold when FGF4 is removed or O2 is shifted to 2 %. Our early studies used very high doses to show maximal enzyme response followed but more recent studies have focused on pathophysiologically relevant doses of single stressors that runt growth but do not kill stem cells after the first few hours after stressing naïve embryos or stem cells. These include 200 mM sorbitol and 0.5 % O2. (Modified from Rappolee et al. 2012)
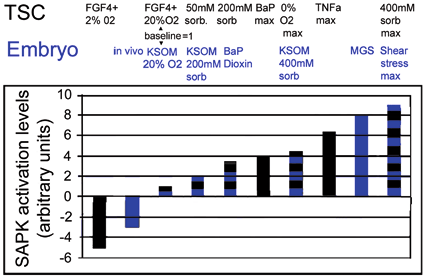
Fig. 4.6
pSAPK activity levels for embryos and TSCs and for multiple stress stimuli and for sub-baseline levels in 2 % oxygen culture and in vivo. Blue shows levels of activated pSAPK induced in embryos in vivo and in culture as well as baseline in standard optimized culture conditions (KSOM/20 % O2). Black shows levels of activated pSAPK induced in TSCs and in culture as well as baseline in standard optimized culture conditions (FGF4 + /20 % O2) as well as reduced stress by emulating the implantation site at 2 % O2 with FGF4 present. What is the significance of differences in peak SAPK activity induced by different stressors? The highest stress suggests the highest response needed for certain types of stress. (Modified from Rappolee et al. 2012)
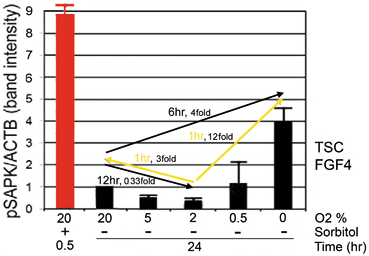
Fig. 4.7
The “direness” index corroborates stimulation indexes, showing that 2 % O2 is the least stressful dose and departure from this requires the fastest changes in the program of the stressed stem cell. Red shows the maximal pSAPK activity stimulation indexes from several stresses (hyperosmolar sorbitol at 400 mM, shear stress at arteriolar velocities, microgravity stress) in embryos and/or TSCs cultured at 20 % O2. Black shows the U-shaped stress response of TSCs to oxygen levels from 20 to 0 % O2. Yellow shows the fastest time (direness index) required to reach a new pSAPK activity levels after switch from one O2 level (tail) to a new O2 level (arrowhead). The fastest times occur when O2 is switched away from 2 % O2. Note that media used in switch were pre-equilibrated for 24 h under the new O2 level. Over this oxygen range AMPK activity produces an S-shaped curve with highest activity at O2 < 2 %, Here where AMPK and SAPK both have maximal activities, maximal responses occur
Embryos exposed to stress in culture undergo cell death, metabolic reprogramming, and genetic and epigenetic reprogramming in an attempt to re-establish cellular homeostasis (Leese 2012; Thompson et al. 2002). Culture stress leads to an increased need for ATP and embryo stage-specific increases in pyruvate or glucose uptake to maintain necessary embryonic events while also mounting responses to the stress. In human embryos glucose is taken up before compaction and mostly used, at least in vitro, through glycolysis to produce lactate (Butcher et al. 1998). By the blastocyst stage, glucose uptake is consumed in greater quantities and converted to lactate. However, most ATP production is via aerobic respiration (Houghton et al. 1996). But, by the late blastocyst, mitochondrial function, as indicated by mitochondrial membrane charge, occurs in mural but not polar TE (or ICM, while overall the trophectoderm is far more active than the ICM in producing ATP. Moreover, the rapidly proliferating polar TE cells near the ICM have mitochondrial inactivity maintained by FGF4 synthesized by the ICM (Chai et al. 1998; Rappolee et al. 1994). It was recently shown that FGF4 decreases mitochondrial activity and increases aerobic glycolysis in cultured TSCs at all O2 levels (Xie et al. 2013b; Rappolee et al. 2011a). FGF4 maintains mitochondrial inactivity in cultured TSCs through tyrosine phosphorylation that inactivates cytochrome oxidase subunit I (Samavati et al. 2008) which is required for activation of ATP synthase as the final step of the electron transport chain. FGF4-dependent tyrosine phosphorylation of the embryonic form of pyruvate kinase (PKM)2 (Xie et al. 2013b) is consistent with maintaining this terminal enzyme in glycolysis in the non-homotetramerized state. When not homotetramerized this enzyme converts phospho-enol pyruvate to pyruvate at a lower rate, thus decreasing ATP production from glucose and depriving mitochondria of pyruvate while increasing carbon availability for proliferative anabolism (Hitosugi et al. 2009; Christofk et al. 2008). Moreover, mouse TSCs maintain high proliferation rates through aerobic glycolysis but use this least efficient means of ATP production. This anabolic mechanism requires highest inputs of glucose to maintain sufficient ATP, carbon and reducing power to sustain high proliferation rates occurring from implantation through gastrulation. However, this metabolic strategy to maintain proliferation of these TSCs is inflexible in that stem cells in the FGF4 response field favour ATP generation by glycolysis over mitochondrial oxidative phosphorylation and would be highly susceptible to perturbation by stress at implantation.
4.2.4 Using Stress Responses to Optimize IVF
In short, we need to reduce stress during embryo handling and culture (Leese et al. 2008; Baumann et al. 2007), and use the stress response to identify normal windows of sensitivity (Rappolee et al. 2013). Windows of sensitivity are created when energy consumption is increased by stimuli that are not part of the normal program (i.e. stress) and compounded when the anabolic program of the stem cell is fixed in a state that is inefficient in producing energy (as discussed in the previous section). Since stress adds to the energy burden of the embryo, periods of high energy demand such as zygotic genome activation, another large wave of zygotic transcription at compaction, cavitation, and implantation restrict the energy available for the stress response . Thus peak energy consumption alone can create a window of sensitivity, but when FGF4 also limits the ability of TSCs to adopt a more efficient mechanism for producing ATP, this enhances stress sensitivity windows even further.
We also need to identify responses to elevated stress that can have negative long-term outcomes (Xie et al. 2011; Rappolee et al. 2011b), in order to optimize embryo culture, and choose the least-stressed embryos for transfer. For example, for all stresses we have studied, whether producing an S-shaped curve or a U-shaped curve for the stress enzyme dose response in TSCs or embryos (Fig. 4.7), cell growth is inversely proportional to stress enzyme activity levels (Xie et al. 2011; Rappolee et al. 2013). For example, TNFalpha, hyperosmolar stress, and benzopyrene produce an S-shaped SAPK activity dose response, but a U-shaped dose response for oxygen levels from 20 to 0 % (Fig. 4.7). Moreover, for growth TNFalpha, hyperosmolar stress and benzopyrene produce a reverse S-shaped curve and oxygen an inverted U-shaped curve. Thus, for all stresses we have tested to date, stress is inversely related to growth and near the dose where stress diminishes stem cell proliferation and population expansion stress also induces stem cell differentiation (Mansouri et al. 2012). There is an inverse relation between SAPK activity and embryo development (Wang et al. 2005). Thus cell number in embryos after IVF/ART, compared with age-matched embryos ex vivo, is a good marker of stress. With improvements in culture media, reduced oxygen tension, and using microfluidics culture embryos have ~ 80 % of the cell number compared with embryos in vivo (Heo et al. 2010; Yang et al. 2014a). Table 4.2 lists factors which affect increases in cell number of embryos during culture. A key conclusion is that many stressors in vivo or in vitro reach a threshold level that diminishes stem cell growth in a clinically relevant range of doses for that stressor. For example for cultured TSCs benzopyrene representing the dose produced by a 2 pack-a-day habit leads to loss of ID2 (Xie et al. 2010), and for oxygen, a combination of a pregnancy at the altitude of Denver (1 mile) with existing maternal hypertension, would lead to significant loss of ID2 and gain of Hand1 (Xie et al. 2014; Yang et al. 2014b). In addition, stresses come in groups and stresses “collide” (Awonuga et al. 2013; Wale and Gardner 2013) such that negative outcomes are exacerbated. An important need indicated by this, and considering the very nature of stressors such as maternal hypoxia, malnutrition, stress hormones, and inflammatory stressors is that we test whether stressors work together to diminish stem cell growth in an additive or synergistic manner.
It is possible that tests for optimal oxygen levels for embryo culture produced a false negative at 2 % (Feil et al. 2006) because the media could not sustain the initial, high anabolic and growth rates that this oxygen level may have produced in stem cells in the embryo. This is likely in considering knowledge on the oxygen-dependent stress enzyme activity and TSC growth optima. The U-shaped curve for the stress response to oxygen levels, shows that optimal potency of TSCs occurs at the same 2 % oxygen levels as the peak stem cell growth rate, but that stress increases and growth decreases upon departure from 2 % in either direction (Zhou et al. 2011; Xie et al. 2013b). That is, SAPK activity is minimal at 2 % oxygen but increases as oxygen rises to 5 % or 20 % or as oxygen decreases to 0.5 % or 0 % (Xie et al. 2014; Zhou et al. 2011; Yang et al. 2014b). Both SAPK and AMPK activity are at near maximal levels within 1 h after switch from 2 %, whereas other switches in oxygen tension from 20 % downwards to any level activate peak stress enzyme levels after 6–12 h (Xie et al. 2014; Zhou et al. 2011; Yang et al. 2014b). This experimental approach provides a way to optimize oxygen and other potential stressors while minimizing stress and maximizing growth rates. Since optimal oxygen supports stem cell growth which rapidly depletes media of nutrients it may be necessary to recalibrate the flow rates of microfluidics devices (Heo et al. 2010) to produce growth rates near those for embryos in vivo. Microfluidics devices provide a nanotechnology where user controls flow rates and pulsatility to optimize embryo growth and development. TSCs grow most rapidly at 2 % oxygen, but must be fed at least every 12 h and recent data indicate that stress (AMPK activation) begins to increase between 6–8 h at the optimum at 2 % oxygen. Certainly, optimization of IVF is likely to include microfluidics use and oxygen levels that support highest growth and potency while allowing allocation of stem cell lineages. Although 2 % oxygen is optimal for TSC growth, without changes of media every 12 h, media become bright yellow due to high levels of anabolic lactosis and decreased pH and AMPK is activated to very high levels incompatible with rapid TSC growth (Zhou et al. 2011). But AMPK activity increases to high levels between 6 and 8 h at 2 % oxygen, indicating even more rapid changes are needed to maintain rapidly dividing TSCs (Yang et al. 2014b). It is also likely that the higher generation of lactate at the blastocyst stage (Houghton et al. 1996) reflects the low efficiency of ATP generation during Warburg metabolism (i.e. aerobic glycolysis and decreased mitochondrial membrane charge). High anabolic growth rate—proliferation requiring macromolecular synthesis—in the polar TE (pTE) maintained by ICM-derived FGF4 as the embryo implants is accompanied by Warburg metabolism, aerobic glycolysis and decreased mitochondrial activity). If IVF moves to more widespread use of blastocyst transfer, it may be optimal to begin phasing in uterine secretory phase products such as glycogen or added glucose during culture since trophectodermal epithelia may phagocytize glycogen so that the blastocyst has sufficient carbon and is acclimatised to the milieu of the uterus at implantation which is hypoxic with O2 concentration at 2 %. In the 1970s and 1980s phagocytosis was used as a marker for trophoblasts but was used more recently to show reversible suppression of differentiated function in the preimplantation blastocyst—((Rassoulzadegan et al. 2000) and citations therein). In those studies FGF4 increased proliferation from the pTE toward the mural trophectoderm (mTE) pole and decreased phagocytosis toward the mTE away from the pTE pole. Glycogen is secreted by the maternal endometrial epithelium and taken up by the trophectoderm (Coan et al. 2005; Burton et al. 2010). It is also likely that glycogen synthesized during oogenesis sustains the embryo during preimplantation development .
4.2.5 Insights from Knockouts of Genes that Regulate the Stress Response
Two major stressors important to gametes, embryos, and stem cells are heat and hyperosmotic shifts in their milieu (Xie et al. 2013b). Maternal and paternal Heat Shock Factors (HSFs) TFs are important protective factors during heat stress as illustrated by developmentally regulated expression of Hsp70.1 (heat shock proteins/HSPs) observed when the zygotic genome activation occurs during culture of unstressed 2-cell embryos (Le Masson and Christians 2011) (Table 4.1). Hsp70.1/Hspa1b is regulated by HSF1 in unstressed oocytes and embryos. But stressed blastocysts (Mezger et al. 1994) require HSF2 to regulate HSPs differentially (i.e. upregulating HSP70.1) to mediate stem cell survival at the blastocyst stage. Moreover, HSF2 is not required for blastocyst survival in a normal vivarium in vivo (McMillan et al. 2002), suggesting that it is required only during elevated stress. This suggests that stem cells arising at the blastocyst stage require HSF2 to resist stress in vivo and in vitro. Cultured preimplantation embryos also require HSPs to resist the stress of arsenite exposure (Summers and Biggers 2003; Krininger et al. 2002; Hansen et al. 2001). Arsenite induces different forms of HSP90 and a phenotypic variation of other HSP isoforms that mediate adaptation to culture. In addition CHOP10 (C/EBP homologous protein-10, aka growth arrest and DNA damage/GADD153, DNA damage inducible transcript/DDIT3) and HSP70 are induced. Heat-induced HSPs are especially protective against stress occurring after compaction in bovine embryos although apoptosis develops and normal increases are diminished after heat shock. The significance of this is that the function of stem cells between the day before, until a week after, implantation in mouse is to grow exponentially (McLaren 1976). Presumably exponential growth is part of the strategy of all mammals during this period, but data from many stressors suggests that any stimulus that diminishes cell proliferation leads to compensatory differentiation in the peri-implantation period. However, arsenite induced apoptosis in embryos before and after compaction occurs even after a conditioning heat exposure given before compaction. This suggests that despite the common outcome of apoptosis which would induce compensatory differentiation, arsenite activates HSP-independent pathways in blastocysts. HSP90 is not necessary for survival of the preimplantation embryo in a normal vivarium but is necessary for placental syncytiotrophoblast function at the maternal interface of the labyrinthine placenta—which is the gas and nutrient uptake surface of the fetal placenta [Table 4.3, (Voss et al. 2000)]. Ostensibly when the exchange cannot survive during hypoxia or cannot produce the secreted angiogenic factors such as VEGF that are the adaptive labyrinthine response, the conceptus fails to develop due to lack of sufficient HSP90-mediated stress response mechanisms. (Rappolee et al. 2013).
The duality of advantageous and disadvantageous functions of SAPK is part of the central dual nature of the stress response (Fig. 4.4). Surviving stress and placing parental genes in the F1 and F2 generations is the ultimate adaptive response of stress enzymes in early stem cells. Higher stress exposures can make the enzymatic response necessary but lower exposures or novel, or “non-physiological”, stressors like some in IVF (e.g., microgravity) may activate stress enzymes that have not been selected for these responses during evolution. Surviving stress may be a product of successful enzyme responses that nonetheless burden future development with epigenetic and imbalanced adaptive responses that are not selective later. If these are late life outcomes they may not negatively affect the offspring reproductively but affect later quality of life after the period of fertility. In most and least stressful media (Xie et al. 2006a) SAPK dual functions are similar to that of TRP53/MDM2 (Jin et al. 2009; Chandrakanthan et al. 2006). In some genetic backgrounds, loss of TRP53 function in null mutant blastocysts, such as inhibition of SAPK in wild type embryos, enhances survival in less stressful media that activate lower SAPK activity levels (Jin et al. 2009; Chandrakanthan et al. 2006) (Table 4.1). Maintenance of low TRP53 function by PI3K also increases survival (Jin et al. 2009; Chandrakanthan et al. 2006) (Table 4.1), Like SAPK activity, TRP53 is higher due to culture stress than in age-matched embryos in vivo (Wang et al. 2005), suggesting that these increases in TRP53 and SAPK activity levels are part of adaptation to culture stress. But for some stressors that activate the lowest levels of stress, SAPK may “over-react” and slow embryo development in vitro. SAPK regulates TRP53 in somatic cells and these may be part of an adaptation to stress exposures during culture. But the interaction of SAPK and TRP53 and their causal effects during culture and in postnatal life has not been studied in preimplantation embryo culture.
Some studies of null mutants have been carried out that were intended specifically to test stress response mechanisms in early embryos but the discovery of the essential function of the elongation initiation factor-α (eIF2α) during preimplantation development was a serendipitous finding. In response to stress new protein translation is curtailed. This is mediated by eIF2α which is phosphorylated by four kinases downstream of (1) dsRNA viral infection (PKR/protein kinase RNA), (2) ER stress (PERK/PKR-like ER kinase), (3) amino acid starvation (GCN2/general control non-derepressible), and a (4) heme deficiency which leads to hypoxia (HRI/Heme-Regulated Inhibitor) (Wek et al. 2006; Proud 2005). When phosphorylated, eIF2α represses translation and leads to ribosome disassembly and storage of mRNA in HuR-associated stress granules instead of mRNA degradation. HuR is also necessary for placentation, as is the ER stress sensing enzyme IRE1 (Table 4.3 Fig. 4.3) This is an important, energy-conserving feature of the re-use of mRNA from the pre-stress program once the stress subsides (Farny et al. 2009). In other words the mRNA program from the unstressed stem cells is stored and reused once the stress subsides, saving the energy required to destroy and re-synthesize mRNA.
Two phosphatases, Ppp1r15a (Protein phosphatase 1 subunit regulatory subunit 15A; stress-inducible) or Ppp1r15b (Protein phosphatase 1 subunit regulatory subunit 15B; constitutively expressed), show redundancy of function in dephosphorylating eIF2α because alone, neither null mutant is lethal (Table 4.3, Fig. 4.3). Deletion of both Ppp1r15a and Ppp1r15b completely blocks eIF2α de-phosphorylation, leaving phosphorylated eIF2α active and enabling continuing block of translation. The dephosphorylation of eIF2α is an important feedback regulation mechanism for maintaining the homeostasis involving phosphorylated-(p)-eIF2α and non-phosphorylated-eIF2α under stress conditions that allow the proper slowing of translation mediated by phosphorylated eIF2α and then resumption of protein synthesis after its dephosphorylation. Both eIF2α and p-eIF2α are physiologically essential and using point mutations of eIF2α in the phosphorylated or de-phosphorylated state cause embryonic lethality (Harding et al. 2009). The eIF2α serine 51 mutant that cannot be phosphorylated under stress conditions, rescues the lethal phenotype caused by deletion of both Ppp1r15a and Ppp1r15b. Thus in the knockout mice, it is the function of the persistent phosphorylated eIF2α that leads to lethality in the double phosphatase knockout blastocysts. This suggests that the blastocyst responds in a normal vivarium to some form of stress that phosphorylates eIF2α, blocking protein translation, and that this function must be reversed to allow continuing embryonic development. This elegant study provides a foundation for further work to discover whether some phases of IVF/ART, particularly embryo collection and culture of preimplantation embryos which (Abraham et al. 2011) activate the ER stress response. The arm of the ER stress response that mediates the eIF2α response is governed by Eukaryotic translation initiation factor 2-alpha kinase 3, (aka PRKR-like endoplasmic reticulum kinase, aka PERK). Altogether there are three arms of the ER response (Mansouri et al. 2012), but the other arm important in early development is the one regulated by IRE1. IRE1 becomes essential for creating the exchange surface of the labyrinthine placenta in mouse within 4 days after implantation in a normal vivarium (Rappolee et al. 2013). IRE1 regulates the Unfolded Protein Response (UPR) by upregulating transcription of chaperones. Although IRE1 is expressed and functional before implantation it becomes essential during the hypoxia that we speculate is a normal part of labyrinthine differentiation before the exchange surface becomes fully functional (Rappolee et al. 2013). Interestingly, during IVF/ART procedures, cryopreservation does not activate the ER stress response (Abraham et al. 2011), but handling in ambient media does. For a suite of stress responses incorporating enzymes including IRE1, SAPK and AMPK, an understanding of activity and function is needed to avoid, measure or experimentally or clinically manage the stress response.
Mekk4 is the MAPK pathway enzyme upstream and necessary for the activation of SAPK and Oct1 is a transcription factor required for the TSC lineage (Xie et al. 2011; Mansouri et al. 2012) (Fig. 4.3). Null mutants embryos for either MEKK4 or Oct1, have more severe and earlier effects in culture than in vivo [Table 4.1, (Abell et al. 2009; Sebastiano et al. 2010)]. In both types of null embryos, polar trophectoderm and proliferating TSCs form in vivo but do not sustain high proliferation of stem cells nor normal differentiation. The null conceptus for both genes dies several days after implantation. In culture, TSC can be isolated from MEKK4 embryos but cannot be sustained for many passages and Oct1 null TSCs cannot be isolated from E3.5 blastocysts. Why are MEKK4 and Oct1 function needed to sustain the TSC lineage in culture? MEKK4 is necessary to mediate the induction of stress enzymes p38MAPK and SAPK. Apparently, both MEKK4 null TSC and wild type TSC cultured with SAPK inhibitors exhibit accelerated epithelial-to-mesenchymal transition (EMT). However this experiment was performed in the presence of FGF4 and 20 % O2 which produced contradictory signals (Zhou et al. 2011). FGF4 increases potency and proliferation and 20 % O2 decreases both features, thus contradicting each other’s functions. Although it was claimed that FGF4 alone sustained high SAPK activity (Abell et al. 2009), removal of either FGF4 or a change from 20 to 2 % O2 with FGF4 still present decreased SAPK activity (Zhou et al. 2011). Thus it is likely that contradictory signals of 20 % O2 and FGF4 together, increase SAPK activity and lead to culture conditions that support slow doubling rates (Fig. 4.6). We have shown that SAPK adapts stressed embryos and TSCs to culture stress (Xie et al. 2011). But, MEKK4 nulls cannot activate SAPK to mediate adaptation to the stress of the contradictory effects of FGF4 and 20 % O2. Similarly, Oct1 has been shown to be necessary for inducing hundreds of stress response genes in fibroblasts prior to the initiation of stress but following phosphorylation regulates the stress response once initiated (Kang et al. 2009a; Kang et al. 2009b). If Oct1 is also required for the setup and activity of the TSC response as in fibroblasts, stressful culture conditions may be one reason for the inability to isolate and culture oct1 null TSCs. There are a number of other insufficiencies in Oct1 null embryos such as their inability to sustain high levels of Cdx2 and Sox2 (Sebastiano et al. 2010), both of which are required to sustain the TSC lineage (Strumpf et al. 2005; Adachi et al. 2013; Avilion et al. 2003). To identify the role of oct1 and Mekk4 in maintaining TSC it is important to attempt to isolate TSC from null blastocysts under less stressful culture conditions, such as FGF4 at 2 % O2. It will also be important to test whether stress in vivo causes an earlier lethal phenotype in oct1 and Mekk4 null embryos than occurs in these nulls in a normal vivarium and to implant oct1 and Mekk4 null embryos into wild type females to isolate the early defect in a stressed vivarium to the inability of null embryos to adapt. This sort of testing has been done for Prolactin-like protein A (PLPA); this angiogenic hormone is not necessary in a normal vivarium but becomes, essential when gestation is stressed by hypoxia [Table 4.1, (Ain et al. 2004)].
Taken together several knockouts that are lethal in normal vivaria suggest that stress is a normal phenomenon but occurs especially during specific periods of development. At the blastocyst stage, enzymes essential for normal stress responses are glutamine synthetase and the Ppp1r15a/b phosphatases which suggest that ammonium stress is a normal occurrence (in vitro and in vivo) and ER stress is normal by the blastocyst stage (Table 4.3). Ammonium is produced under normal conditions but causes stress if not sequestered. For glutamine synthetase, there is the additional lesson that a confounding hyperoxic stress in vitro prevents its function in responding to normal stress and this leads to morbidity. After implantation there are several stress response genes that must act to sustain the response to normal hypoxia by the epithelium of the mouse labyrinthine placenta (aka human villous placenta) which mediates gas/nutrient exchange and hormone secretion. These include p38MAPK, HIFs, HSP90, HuR, and IRE1 which were reviewed previously (Rappolee et al. 2013) (Table 4.3). These stress response genes may also be required for earlier events during the preimplantation period, but phenotypes are masked in null mutants by residual maternal mRNA and proteins. The need for these genes may also be revealed by stressing preimplantation development in vivo. Gestational stress reveals a second class of knockout lethalities for genes that mediate stress responses but are needed, in vitro or in vivo, only when stress is elevated above normal levels. These include HSF2 and MEKK4 (Table 4.1) which are not required normally but become essential, as with earlier phenotypes, when stress is applied to the blastocyst (HSF2) or TSCs (MEKK4). PLPA is a powerful example of a post implantation gene mediating a hypoxic stress response that becomes essential only during a hypoxic, stress gestation.
4.2.6 Stress Enzymes in the Survival Response of Stem Cells and Embryos
Arsenite, as well as hyperosmolar stress and heat shock, induces AMPK activity in the oocyte and this enzymatic activity is necessary for oocyte maturation during elevated stress (LaRosa and Downs 2006, 2007). It was initially suspected that AMPK would play a role in mediating the response to stressors which cause the decrease in ATP production and increase in AMP and that this would require a shift from anabolism to catabolism to lower energy consumption (Leese 1998, 2002). Later, pioneering work of the Down’s lab showed that AMPK is activated by many stresses in oocytes and that anabolism was decreased in an AMPK-dependent manner (LaRosa and Downs 2006, 2007; Chen et al. 2006), although mechanisms mediating stress–induced oocyte maturation were not tested. Hyperglycemia as well as insulin resistance accompanying diabetes have negative effects on embryo development and pregnancy. The level of AMPK is decreased in both oocytes and blastocysts under conditions of insulin resistance (Louden et al. 2008; Ratchford et al. 2007). A large part of the negative effect of insulin resistance on embryo quality can be reversed by re-introduction of AMPK activity into those affected embryos (Eng et al. 2007). This mediates gamete survival by slowing anabolism and increasing catabolism, but also mediates organismal survival in producing a fertilizable oocyte ready to produce an embryo. This will be discussed in the second part, along with more recent experiments elucidating the roles of AMPK in organismal survival through stress-induced differentiation .
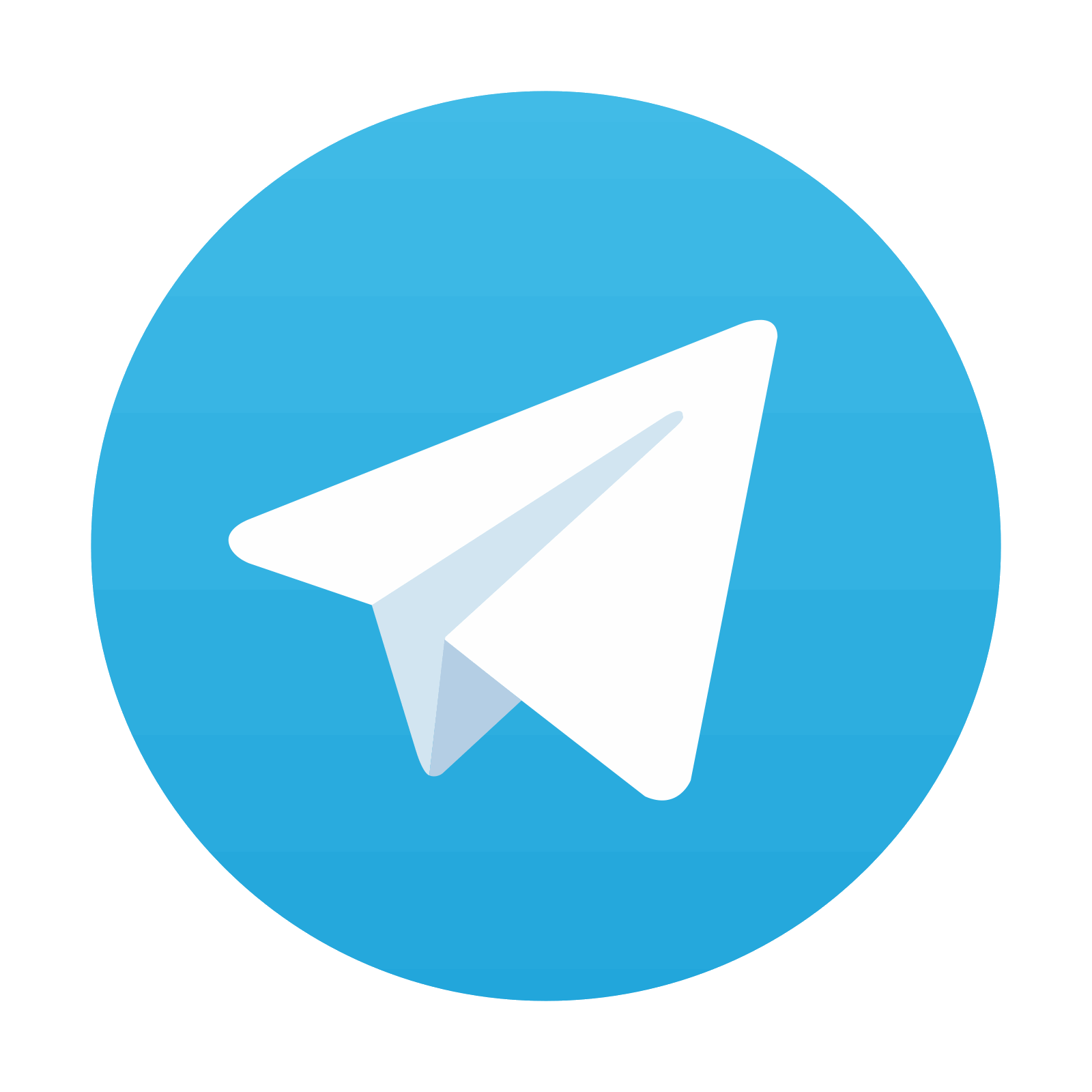
Stay updated, free articles. Join our Telegram channel
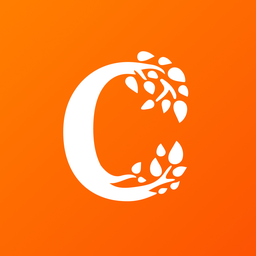
Full access? Get Clinical Tree
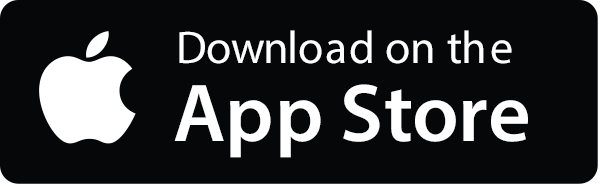
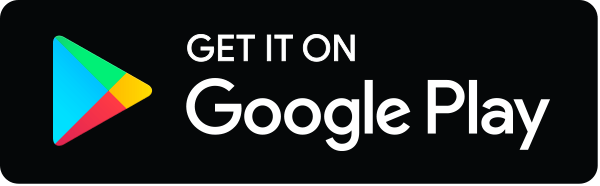