Molecular Biology of Bone Tumors
During the past two decades, our knowledge of the molecular events that are responsible for the development and progression of many human neoplasms, including those that affect the skeleton, has dramatically improved. This has been made possible by numerous technologic developments that have contributed to the understanding of cellular biology and that shed light on some molecular aspects of the malignant transformation of cells. The past decade is defined as the postgenomic era, in which many diseases, including bone tumors, are beginning to be understood in the context of genome changes rather than only in relation to individual genes and their respective pathways. This is due to the completion of the sequencing of the human genome released to the public as the so-called reference human genome sequence in 2004.9,10,23 This accomplishment has altered the landscape of biomedical science including medicine and has changed the environment in which health care is provided.7,3,20,23,27 It also has accelerated improvements in our understanding of skeletal physiology as a complex body-supporting organ and has provided new insights into the biology of bone tumors.
In this chapter, we focus on some of the most promising areas of molecular research related to the understanding of the molecular pathogenesis of bone tumors and that are relevant to their diagnosis, as well as representing novel, still to be fully explored, therapeutic targets. The pathogenesis of neoplasia cannot be understood without basic knowledge of the biology of the cells involved in the process of transformation. Therefore the description of the molecular biology of bone tumors is preceded by a section describing some fundamental techniques used to investigate the function of cells and on the molecular biology of physiologic bone formation. The description of both skeletal physiology and the biology of bone tumors is restricted to a few fundamental aspects of cell function in physiology and disease. An interested reader is referred to comprehensive textbooks concerning the biology of the skeleton as well as the biology of cancer. The two recommended highly respected and periodically reviewed texts are by JP Bilezikian, et al, Principles of Bone Biology, and by RA Weinberg, The Biology of Cancer.
Molecular Techniques
The most frequently used techniques to elucidate the genetic abnormalities discussed here are based on the extraction and analysis of deoxyribonucleic acid (DNA), ribonucleic acid (RNA), or proteins. To facilitate the separation and identification of the genetic material, long strands of DNA are usually cut with specific restriction enzymes (endonucleases). Restriction endonucleases are a unique class of enzymes that recognize specific nucleotide sequences and cut double-stranded DNA at specific points. These enzymes are used in nearly all gene reconstruction and gene transfection (gene transfer) experiments, collectively known as DNA recombinant technology or genetic engineering. The many techniques used to identify normal or altered components of a genome are assembled into strategies capable of identifying both the structural and functional aspects of the genetic element under the study.
The techniques of modern molecular genetics can be divided into three main groups: DNA hybridization techniques, technologies that involve polymerase chain reaction (PCR), and genomic sequencing with high throughput genomic profiling. Commonly used terms and techniques are described in the following paragraphs.
Hybridization Techniques
Hybridization techniques were originally designed to identify individual components of our genetic material such as genes and expressed species of RNA such as mRNA or proteins.11,25 In general, the material is first separated by gel electrophoresis and transferred to a membrane; then the specific DNA, RNA, or proteins are identified with a detection probe. These techniques involve Southern blotting, in which specific components of DNA are identified, Northern blotting, in which specific species of RNA are analyzed, and finally Western blotting for the identification of specific proteins. These techniques are described here for historical purposes. However, today Southern blotting is rarely used, most often in the identification of the ablated regions of the genome in the development of genetically engineered animals. Northern blotting has almost been completely eliminated from the modern armamentarium of molecular techniques and has been replaced by more effective techniques such as PCR and quantitative PCR. On the other hand, Western blotting remains the most popular technique used in molecular biology and pathology for diagnostic clinical workups.
Southern Blotting
Southern blotting is used to identify particular sequences within genomic DNA. Traditionally, DNA is digested with restriction endonucleases, and the resulting DNA fragments are separated by size using electrophoresis through agarose gels. After in situ denaturation of the fragments by an alkaline solution or heat, the DNA is transferred from the gel onto a solid support (nylon membranes, nitrocellulose filters). The relative positions of the fragments are preserved during this transfer. Subsequently, the DNA blotted to the solid support is hybridized with a known, specific DNA or RNA probe with a radioactive label and is visualized by autoradiography to identify positions of bands that are complementary to the probe.
Northern Blotting
Northern blotting is used to determine the size and amount of specific messenger RNA (mRNA). As in Southern blotting, the RNA is separated by electrophoresis on the basis of size using denaturing agarose gels and is transferred to a solid support (nylon membranes, nitrocellulose filters). The RNA of interest is located by hybridization with an appropriately labeled DNA or RNA probe.
Western Blotting
Western blotting is used for the immunologic identification of proteins extracted from tissues or cells. For this procedure, protein extracts are separated by electrophoresis and then transferred to a solid support and identified with labeled or unlabeled antibodies that recognize the antigenic epitopes displayed by the target protein (Fig. 3-1). When unlabeled antibodies are used, the identification of the bands is done with a labeled secondary antibody to the immunoglobulin class of the animal species in which the first antibody was raised. In general, the Western blotting procedure depends on three key steps: (1) the separation of proteins by size using gel electrophoresis, (2) the transfer of separated proteins to a membrane, and (3) the detection of a target protein by specific antibodies. Typically, the antibodies used in Western blotting recognize a short specific linear sequence of amino acids within the target protein. The target protein is visualized as a band on a blotting membrane using radiography or an imaging system with secondary antibodies labeled with chemiluminescence or fluoroluminescence. The thickness of the band or, in general, the staining intensity corresponds to the amount of the target protein. Western blotting is one of the most efficient and popular techniques, utilizing simple equipment and inexpensive reagents.
Polymerase Chain Reaction
PCR is an in vitro technique used to amplify a DNA segment lying between two regions of known sequences by using two oligonucleotide primers.5,8 The primers are complementary to the sequences on the opposite strands of the target template DNA and flank the DNA segment being amplified. The reaction mixture contains the four nucleotides required for DNA synthesis in the presence of a heat-stable DNA polymerase. The procedure comprises three basic steps: heat denaturation of the DNA, cooling and annealing of the primers to their target sequences, and extension by a heat-stable DNA polymerase (Fig. 3-2). Because the product generated in the first PCR round serves as the template for subsequent rounds, an exponential amplification of the DNA segment of interest may be obtained by repeating the cycles. The usual amplification level is on the order of 106 after 25 to 30 cycles. Greater amplification (109 to 1011) of DNA copies may be achieved by repeating the cycles with the product generated in the first run used as a template and fresh DNA polymerase. PCR can also be used to generate amplified DNA from mRNA after initial reverse transcription.
Real-time reverse transcription polymerase chain reaction (RT PCR), or quantitative polymerase chain reaction (qPCR), is based on the concept of standard PCR and facilitates the quantitative assessment of a template DNA (Fig. 3-3). It utilizes fluorescent dyes tagged to a probe, which anneal to the amplified product; the signal intensity is proportional to the amount of DNA being amplified.1,14,29 RT PCR utilizes the standard PCR sequence, specific forward and reverse primers, and a fluorescently labeled probe that provides quantitative assessment of the template DNA. In general, the intensity of the fluorescence emitted during the amplification cycle correlates with the amount of the DNA that is amplified. Fluorescence intensity increases proportionally to the amplification cycles; after a few initial cycles it surpasses a threshold level set above the background and starts to increase exponentially. The Cq value (quantification cycle), which represents the number of PCR cycles that pass before the threshold is reached, is a measure of the original template DNA. By comparing the results of samples of unknown concentration with a series of standards, the amount of template DNA in the measured sample with unknown concentration can be determined. RT PCR has wide applications in gene expression analysis, pathogen detection, and genetic testing. It can also be performed in a multiplex form, assessing the expression level of many genes.
Genomic Sequencing
With the completion of the Human Genome Project, the release of the first draft of the human genome sequence in 2001, and the final publication of a reference human genome sequence in 2004, genomics became a mainstream discipline of biomedical research.9,10 The rapid advances in genomic sequencing technologies facilitated the ability to sequence the genome of an individual human being in 2008, offering genomic profiling of tumor samples and their paired germ line DNA to cancer patients.12,16,19,21,24,28 This approach has provided new insights into the biology of many diseases, including cancer, potentially improving human health. Technologic development is the driving force of genomics and is responsible for the invention of new methods, reagents, and instruments.23,24,30 It also inspires the improvement of efficiency and output, with consistently decreasing costs making genomic profiling possible for individual patients (Fig. 3-4). The novel genome sequencing technologies collectively referred to as next-generation sequencing enable assessment of mutations in several hundreds of potential therapeutically targetable genes and are also capable of applying total genome sequencing to individual patients. It is predicted that during the next decade we will witness an unprecedented genomic characterization of many human cancers. This will be accomplished in parallel with a more in-depth understanding of the biology of our genome and how it functions in a disordered state in cancer development and progression. Genomic approaches are also anticipated to open novel avenues for the improvement of cancer therapy and the overall effectiveness of cancer patient outcomes. The most advanced genome sequencing technologies are capable of analyzing the entire human genome sequence within a few days at reasonable cost (Fig. 3-5). Such technologies offer the detection of a full spectrum of genomic abnormalities that include point mutations, copy number alterations including homozygous and hemizygous deletions, copy gain, translocation breakpoints, and pathogen identification.
High Throughput Genomic Profiling
The completion of the Human Genome Project and the knowledge of the complete sequence of our genome enabled the development of a full roster of high throughput genomic platforms that facilitate the analysis of various structural and functional aspects of our genome both in physiology and disease. They include the single nucleotide polymorphism-based, high density microarrays with which we can assess precisely the copy number change of specific genomic regions and analyze it on a global genomic scale.6,15,27 The expression patterns of genes can be investigated by testing the levels of mRNA using the cDNA microarray.4,15 Similarly, the expression levels of various regulatory RNA species such as microRNA can also be assessed on a total genome scale.22 Finally, the epigenetic modifications of the genome, including methylation and histone acetylation, can be analyzed with specific strategies and microarrays.18 The genomic high throughput platforms are complemented with proteomic and metabolomic technologies that are capable of analyzing the expression profiles and status of metabolic activities in the entire cellular proteome.2,13,17,26 All of these platforms are capable of generating tsunami waves of data that represent a major analytical challenge to the health care system in our time.
Molecular Biology of Bone Formation
The human skeleton is a complex body-supporting organ with more than 200 bones adapted to bipedal locomotion. The majority of the human skeleton is formed by endochondral ossification, which is responsible for growth and patterning of the long bones of the limbs and elements of the axial skeleton (Fig. 3-6).60,84 Fewer bones, predominantly the flat bones and the clavicle, are formed by intramembranous ossification.60,84,112 Endochondral ossification starts as condensations of mesenchymal cells that form cartilaginous templates subsequently remodeled into bone. The condensation centers have peculiar zonal patterns of differentiation in which the central component differentiates into cartilage while the peripheral cells form perichondrium, which differentiates into osteoblastic precursors and in the mature phase forms the periosteum. The central components of the cartilage template undergo stepwise differentiation into hypertropic chondrocytes.70,73 This maturation process requires cessation of proliferation. Hypertropic chondrocytes, after exiting the cell cycle, die by apoptosis and their cartilage matrix is used as a template for bone formation. This process requires precise zonal orchestration of two main cell lineages involved in skeletal development—chondroblasts and osteoblasts—which are mediated in this process through Indian hedgehog (IHH) signaling.52,53 In intramembranous ossification, flat bones develop directly from mesenchymal condensation and no interaction with a preformed cartilage template is needed for bone development. During the past decade, there has been a dramatic development of our understanding of the molecular mechanisms that orchestrate these processes. The genes and their respective proteins that play a role in skeletal development can be, in part, used as biomarkers for differential diagnosis of bone tumors and can provide new insights, not only into the physiology of bone, but also on the pathogenesis of bone tumors.
Three distinct cell lineages of mesenchymal origin participate in the formation of the skeleton: osteoblastic, chondroblastic, and osteoclastic.74,85 These cells cooperate and influence one another in the formation and remodeling of the skeleton. For example, osteoblasts produce factors that affect the function of osteoclasts. In the enchondral ossification model, the formation of cartilage matrix is a prerequisite event for osteoid deposition in the primary spongiosa. Subsequent resorption of the primary spongiosa by osteoclastic cells is an important step in bone remodeling and formation of the secondary spongiosa. Other factors that regulate bone-forming and bone-remodeling functions of cells are produced in response to extracellular growth factors and hormonal stimulation. It is speculated that the osteoblastic cells participating in intramembranous and enchondral bone formation may be phenotypically distinct or may even originate from another sublineage differentiation pathway.42,64,65,103,107,108 In general, bone development and its maintenance are regulated by conserved pathways linked to the interactive network of growth factors, regulated by hormones, their respective receptors, and vitamins.36,65 The major hormones and their receptors involved in regulating bone development include parathyroid hormone, parathyroid hormone-like peptide, thyroid hormones, and steroid hormones as well as vitamin D.109 In addition to contributing to the development of the skeleton, these factors also control bone mass and bone maintenance during adult life.90,91,100,108,112
The unique capacity of bone for complete structural and functional renewal depends on the presence of stem cells that can proliferate rapidly and differentiate into different bone-forming cell lineages. It is postulated that the unique ability for renewal is in the bone itself.104,105 A unique class of matrix polypeptides, collectively designated as bone morphogenetic proteins (BMPs), is implicated as potent early inducers of bone formation and cartilage development, as well as of bone reconstitution after injury.41,44,46–49,66,77,82,87 BMPs also seem to play a ubiquitous role in skeletal patterning, limb development, and organogenesis of some nonskeletal structures.55,58,71,72 They show a significant sequence homology to the beta family of transforming growth factors (TGFs).45–49,51,67
Osteoblastic Lineage
Osteoblastic lineage cells, as they participate in bone production, are separated into several distinctive subgroups on the basis of their gene expression patterns, ability to produce extracellular matrix, and their relationship to bone (Fig. 3-7). The development of osteoblastic lineage cells and bone production are regulated by a complex cascade of growth factors, which are graphically depicted in Figure 3-8.37,38,53,60,61,63,68,69,100,112 Skeletal progenitor cells that co-express SOX9 and RUNX2 are not fully committed to osteoblastic lineage.56,83 It appears that the osteoblastic lineage fate in the early phases of lineage commitment is controlled by the transcription factor Osterix/SP7.54,81,107,110 It belongs to a super family of Kruppel-like zinc-finger transcription factors and is expressed at high levels in osteoblasts. Low levels of Osterix/SP7 are also found in prehypertrophic chondrocytes. The expression level of Osterix is controlled by RUNX2 and is stimulated by interaction with the nuclear factor of activated T-cells (NFAT). RUNX2 is required in early phases of osteoblastic progenitor cells and is downregulated in mature osteoblasts. The activity of RUNX2 is enhanced by multiple factors such as SATB2, ATF/CREB family members, and is reduced by DLX3 and MSX2. The transcription factors SP3 and ATF4 play a role in the late phases of osteoblastic differentiation. ATF4 is expressed at high levels in osteoblasts because of its inhibited proteasomal degradation. The activation of osteoblasts is also modified by phosphorylation of ATF4 by the kinase RSK2 and interaction with SATB2. Finally, the matrix production of osteoblasts is likely controlled by FRA1. Osteocalcin gene upregulation is a feature of late osteoblastic and osteocytic differentiation. Similar patterns of expression in association with later stages of osteoblastic differentiation are seen in reference to osteopontin, galectin-3, and CD44. The production of collagen type I starts with the onset of differentiation and increases with the cell’s progression to a mature osteocytic state.
In summary, there is a reciprocal relationship between two distinct groups of genes that predominantly act on one another in a proliferative and postmitotic differentiated stage. The high levels of histone proteins and other genes related to the proliferative stage gradually approach residual constituent levels or are completely suppressed with the onset of osteoblastic differentiation (see the section in this chapter on cell-cycle regulation). One of the earliest events that signifies the onset of osteoblastic maturation is the upregulation of collagen type I genes, followed by the upregulation of alkaline phosphatase and matrix GLA protein. High levels of osteopontin, osteocalcin, and collagenase are characteristic of the mineralized phase of osteoblastic differentiation.
Chondroblastic Lineage
A number of ubiquitous growth factors have been shown to mediate early phases of skeletal development.68,84 Their role in the regulation of skeletal growth and cartilage formation is graphically depicted in Figure 3-8.61,69,73,76,78,80,82,99 One of the transcription factors that acts very early and is responsible for rescuing of pluripotential mesenchymal cells into skeletal progenitor cells is SOX9.83,107 SOX9 is a member of the SOX gene family of transcription factors characterized by high-mobility-group box DNA binding motif showing homology with sex determining factor SRY. Skeletal progenitor cells with upregulated SOX9 and RUNX2, a Runt domain transcription factor, are capable of bimodal differentiation into chondrocytes and osteoblasts. The chondroblastic fate of the skeletal progenitor cells is ensured by direct interaction of SOX9 transcriptionally inhibiting RUNX2 and indirectly by the repression of NKX3.2/BAPX1. Collective in vitro and in vivo evidence indicate that SOX9 activity is required for chondrocyte differentiation, but it requires cooperation with additional transcription factors such as PAX1 and PAX9, paired-box family members, and NKX3-2 and NKX3-1, homebox family members. In addition, such transcription factors as RUNX1, DLX5, and DLX6 as well as BARX2 have been implicated in chondrocyte differentiation. SOX9 has been shown to control expression of important cartilage matrix proteins, including COL2A1, COL9A1, COL27A1, and matrilins. Cooperation with additional SOX family members such as SOX5 and SOX6 are required to differentiate chondroblasts into early chondrocytes. The development of fully matured cartilage requires maturation of chondroblastic/early chondrocytic cells into prehypertrophic and hypertrophic chondrocytes. This process is again mediated by SOX genes, which further signifies their central role in cartilage development. Maturation into hypertrophic chondrocytes is accomplished through NKX3-2 mediated downregulation of RUNX2 and the β-catenin/TCF complex. Downregulation of RUNX2, IHH, and FGFR3 plays a role in the timing of the chondrocyte hypertrophic stage. Multiple regulatory loops control the sequential suppression of these factors via the helix-loop-helix transcription factor, TWIST1, and other cofactors, such as GRG5, HDAC4, DLX5, and DLX6. Additional factors such as MSX2, MEF2C, MEF2D, and FRA2 are likely involved in the regulation of the chondrocyte hypertrophic phase. Terminal maturation and progression to apoptosis is not fully understood at this time but appears to be regulated by the leucine zipper protein c-Maf. Chondrocytic cells express the genes such as collagen type II (COL2A1) that are responsible for the formation of the cartilage matrix and the genes that contribute to the formation of the primary proteoglycans in the matrix, which are aggrecan, decorin, biglycan, and fibromodulin.50,52,57,98 These glycoproteins are responsible for the osmotic properties of the matrix and its interaction with collagen II fibers, and provide the matrix with structural integrity.43,62,76,79,88 Extracellular matrix synthesis is in part regulated by C-type natriuretic peptide (CNP) and the MAPK pathway of fibroblast growth factor (FGF) signaling.64,111
Osteoclastic Lineage
Orchestration of skeletal development requires not only bone production, but also resorption of mineralized matrix to achieve functionally optimal patterning of individual bones.40,86,87 The cells in bone that perform this function and are capable of resorbing mineralized matrix are multinucleated osteoclasts (Fig. 3-9). It is generally accepted that osteoclasts develop by means of a differentiation pathway distinct from that of osteoprogenitor and cartilage progenitor cells. Numerous experimental data indicate that osteoclasts are derived from hematopoietic precursor cells present in the bone marrow, spleen, and peripheral blood.91–93,102 They have a common differentiation pathway with macrophages and develop distinct phenotypic features in the final steps of their maturation. These cells, in response to chemotactic stimulation, migrate to areas of exposed matrix where the bone lining cells have retracted and form sealing zones on the matrix by interactions of αvβ3-integrins with matrix proteins. Three of these receptors—, αvβ3 (fibronectin receptor), α2β1 (collagen receptor), and αvβ1—are essential for adherence and subsequent bone resorption. Osteoclasts secrete acid to the sealed pockets on the surface of bone to dissolve the mineralized matrix and protein degrading enzymes to disintegrate exposed matrix proteins. These functions are performed in a specialized apical cell membrane surface referred to as a ruffled border. Only a few biochemical reactions can solubilize mineralized deposits of the bone matrix (i.e., hydroxyapatite), and for these reactions to proceed, the environment must be acidic (low pH).60,106 In fact, sealed bone lacunae are acidic and reach this state by a V-type proton pump that uses adenosine triphosphatases (ATPases) that operate in the ruffled border of osteoclasts. The levels of these enzymes are extremely high in activated osteoclasts. It can be envisioned that without the sealing of the resorptive lacunae, the proton pump is very unlikely to be effective in lowering the pH; thus solubilization of the mineral cannot be accomplished. After solubilization of the inorganic component, the remaining protein material is degraded with the use of multiple proteolytic enzymes such as collagenases, lysosomal proteinases, and cathepsins. There is evidence that osteoclasts can go through several activation-resorption cycles. It is speculated that cessation of bone resorptive activities must be accomplished by programmed cell death (apoptosis).
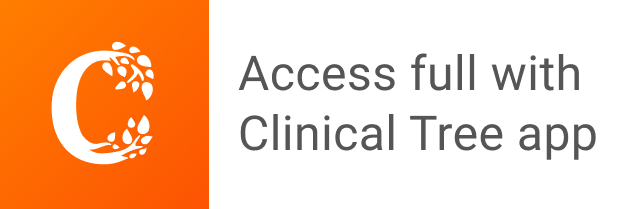