Chapter 14
Microscopic Anatomy of the Zygapophysial Joints, Intervertebral Discs, and Other Major Tissues of the Back
Microscopic Anatomy of the Zygapophysial Joints
Zygapophysial Joint Articular Cartilage
Zygapophysial Joint Articular Capsule
Supporting Cells and Extracellular Matrix of Connective Tissue: Functional Components
Microscopic and Molecular Structure of the Intervertebral Discs
Normal Aging of the Intervertebral Discs and Intervertebral Disc Degeneration
Glycosaminoglycans and Proteoglycans
Microscopic Anatomy of Other Major Tissues in the Back
Much of the current anatomic research related to the spine is concerned with the zygapophysial (Z) joints and the intervertebral discs (IVDs). The gross anatomy of these structures is covered in detail in Chapter 2. The characteristics of these structures unique to the cervical, thoracic, and lumbar regions are covered in Chapters 5, 6, and 7, respectively. Because much of the current investigation related to the Z joints and IVDs has been carried out in the lumbar region, Chapter 7 describes the Z joints and IVDs in significant detail. However, a considerable amount of the research on these two tissues is associated with their microscopic anatomy and molecular structure. The results of these investigations provide a greater understanding of normal, as well as pathologic, structure and function at the microscopic, ultrastructural (electron microscopic), and molecular levels.
Microscopic Anatomy of the Zygapophysial Joints
As with all diarthrodial joints, the articular surfaces that form the Z joints are covered with shiny hyaline cartilage. This cartilage is lubricated by synovial fluid that allows the bones to glide smoothly over each other with minimal friction (Swann et al., 1974). A tough sleeve of dense connective tissue envelops the articular cartilages and joint cavity of the Z joints posteriorly. This connective tissue sleeve is known as the fibrous capsule. Anteriorly the ligamentum flavum takes the place of the articular capsule of the Z joint (Xu et al., 1991). A thin inner layer of highly vascularized connective tissue called the synovial membrane lines the joint capsule. Cells within the synovial membrane manufacture the synovial fluid.
This section discusses the microscopic anatomy of the articular cartilage, capsule, and synovial membrane of the Z joints. A working knowledge of connective tissue is important in treating pain of spinal origin because most tissues involved in the formation of the Z joints (and IVDs) are connective tissue, and pain arising from the Z joints is a significant cause of back pain (Mooney & Robertson, 1976; Kirkaldy-Willis, 1988). Therefore a section on connective tissue, including hyaline cartilage, immediately follows this section on Z joints.
Zygapophysial Joint Articular Cartilage
The articular cartilages lining the superior and inferior articular processes of each Z joint are similar in many respects to the articular cartilage associated with most synovial joints of the body. This means that the articular cartilage lining each of the articular processes of a Z joint is composed of a special variety of hyaline cartilage that is durable, lubricated by synovial fluid, compressible, and also able to withstand large compressive forces (Standring et al., 2008).
The purposes of Z joint articular cartilage are to protect the articular surfaces of the superior and inferior articular processes by acting as a shock absorber and to allow the articular surfaces to move across one another with little friction. Both functions are carried out efficiently. In fact, the coefficient of friction for typical articular surfaces is less than 0.002, which means that the two surfaces of a typical Z joint glide across each other with much greater ease than they would if they were both made of ice (the coefficient of friction for ice sliding on ice is <0.03) (Whiting, 1998).
The articular cartilage of a single Z joint surface is small; in fact, the lumbar articular surfaces measure approximately 8 × 10 mm (Giles, 1992a, b). The Z joint articular cartilage also is approximately 1 to 2 mm thick (Figs. 14-1, 14-2, and 14-3). The concavity of the cartilage on lumbar superior articular facets is thicker than the periphery of the same surfaces. This is the opposite from that typically found in other joints of the body where the concavity of a joint surface usually is lined by thinner cartilage than that surrounding the concavity.
FIG. 14-1 Zygapophysial (Z) joint. A and B, Z joint from a posterior view and a horizontal section, respectively. C, Z joint after magnification by approximately a factor of 10. The articular cartilage, subchondral bone, and articular capsule are prominently displayed. In addition, a Z joint synovial fold is prominent. Notice that the articular capsule has an outer, tough fibrous region. The center of the synovial fold is more vascular and contains adipose tissue. A nerve can be seen passing through this latter region. A synovial lining can be seen on the deep surface of the articular capsule and the synovial fold. The box enclosing much of the synovial fold and a portion of the superior articular process is the region shown at higher magnification in Figure 14-7.
FIG. 14-3 Magnetic resonance imaging scan of the lumbar region performed in a parasagittal plane. The plane of section approximately corresponds to that of Figure 14-2. Notice the intervertebral discs, the intervertebral foramina, and their contents. (Magnetic resonance image courtesy Dr. Dennis Skogsbergh.)
Z joint articular cartilage is made up of 75% water and 25% solids (Giles, 1992a, b) and consists of cells embedded in an abundant and firm matrix (Fig. 14-4). The cells that produce the cartilage matrix are chondroblasts, and in mature cartilage they are known as chondrocytes (Table 14-1). The matrix consists of an intricate network of collagen fibers surrounded by proteoglycans and glycoproteins. The concentration of these constituents of articular cartilage differs from one part of the joint surface to another and also at different depths from the joint surface (Giles, 1992a, b).
Fresh hyaline cartilage is bluish white and translucent. In stained, fixed preparations, the matrix appears glassy, homogeneous, and smooth. Distributed throughout the matrix are spaces called lacunae, and within each lacuna is a chondrocyte. As with all articular cartilage, that of the Z joints has no nerve supply and no direct blood supply. Chondrocytes must receive nutrients by diffusion across the cartilage matrix from several sources. These sources include the blood vessels within the synovial membrane that is located along the peripheral margin of the nonarticular portion of the cartilage, the synovial fluid, and the blood vessels in the adjacent bone (Standing et al., 2008).
Chondrocytes are found singly in the lacunae. Commonly, especially in cartilage that is actively growing, the lacunae are grouped into clusters of two or more. These clusters are called cell nests or isogenous cell groups (Fig. 14-5). The cells within the nests have arisen from the mitotic activity of a single chondrocyte; therefore the presence of isogenous cell groups signifies interstitial cartilage growth. This is supported by electron microscopic findings revealing that the chondrocytes within a cell nest exhibit a well-developed rough endoplasmic reticulum, a Golgi complex, and a large amount of glycogen and lipid.
Articular cartilage differs from typical hyaline cartilage in that the articular surface does not possess a covering of perichondrium (Giles, 1992b; Standring et al., 2008). Instead the cells of the articular surface appear flat and are closer together than they are farther within the cartilage matrix. In addition, the matrix of the articular surface becomes dense and fibrous. The collagen fibers, which course perpendicular to the articulating surface from deep within the cartilage matrix, curve as they reach the joint surface and become oriented parallel to the free edge of the articular cartilage.
Cartilage Matrix
Collagen: The collagen component of hyaline cartilage consists of type II collagen fibers. These fibers are relatively thin and course in all directions within the cartilage. Usually they are not visible with the light microscope because they are masked by another component of the cartilage matrix, the ground substance. The collagen fibers can be seen easily with an electron microscope.
Collagen functions to bind the cartilage together, protect the chondrocytes, allow for attachment of the articular cartilage to the subchondral bone, and help resist compressive loads (Giles, 1992a). Because collagen is an important constituent of all the connective tissue components of the Z joints and the IVDs, it is discussed in detail in Supporting Cells and Extracellular Matrix of Connective Tissue: Functional Components.
Ground substance: Chemical analysis of the ground substance of the extracellular matrix of hyaline cartilage reveals that it contains a small amount of glycoproteins and a high concentration of three types of glycosaminoglycans: hyaluronic acid, chondroitin sulfate, and keratan sulfate. The chondroitin and keratan sulfates are joined to a core protein to form a proteoglycan monomer. These macromolecules interact with the collagen and elastic fibers of the hyaline cartilage matrix (Fig. 14-6).
At one end of each core protein is a hyaluronic acid–binding region (see Fig. 14-6). At this site the proteoglycan units are joined to hyaluronic acid molecules to form long proteoglycan–hyaluronic acid (PG-HA) aggregates. The interaction of the proteoglycan monomer with hyaluronic acid is strengthened by the presence of a link protein (see Fig. 14-6). Proteoglycans and glycosaminoglycans are discussed in further detail later in this chapter with regard to the IVD.
Chondronectin is a glycoprotein found in cartilage. Glycoproteins differ from proteoglycans by their low carbohydrate content, different repeating disaccharide units, and the absence of sulfate esters. Chondronectin participates in the adhesion of chondrocytes to type II collagen. Common glycoproteins found in other body tissues include laminin and fibronectin. Laminin is found in basal laminae and is partially responsible for the adhesion of epithelial cells. Fibronectin is found in blood, plasma, fibroblasts, and some epithelial cells and helps to mediate normal cell adhesion and migration (Table 14-2).
Clinical and biomechanical considerations: Normally fluid moves out of articular cartilage when it is compressed and back into the cartilage when the Z joint is distracted. Such movement may help nutrients diffuse through the matrix to the chondrocytes. Articular cartilage can deform considerably when heavy compressive loads are applied to a joint. However, it returns to its previous state when the load is removed. If injured, articular cartilage heals rather slowly (a 1-mm defect heals in approximately 4 weeks). Passive movement of the joint may stimulate cartilage regeneration, whereas immobility results in the development of adhesions. Intermittent light weight-bearing activity does not stimulate cartilage regeneration but does stop the development of adhesions (Giles, 2005).
Articular cartilage becomes yellow, thinner, and more brittle with age, and undulations that may develop into ragged projections appear as a result of “wear and tear” of the joint surface (Standring et al., 2008). Also with age, fissures or cracks may develop in the articular cartilage. The development of such fissures is known as fibrillation of articular cartilage. The fissures may extend from the joint surface to the subchondral bone.
Zygapophysial Joint Articular Capsule
The Z joint capsules attach to the margins of the opposed superior and inferior articular facets of adjacent vertebrae throughout the vertebral column. The capsules are longer and looser in the cervical region than in the lumbar and thoracic regions. The articular capsule of a typical Z joint covers the joint’s posterolateral surface. It consists of an outer layer of white and shining dense fibroelastic connective tissue with bundles of collagen fibers coursing parallel with one another. Deep to the outer fibrous layer is a vascular central layer that is softer and more extensible than the outer layer, and is made up of elastic fibers, similar to the ligamentum flavum, areolar tissue, and loose connective tissue. The third and deepest layer of the Z joint capsule is an inner smooth and shining layer consisting of a white synovial membrane (Giles & Taylor, 1987; Yamashita et al., 1996). The outer, connective tissue layer of the capsule is tough and is essentially composed of parallel bundles of collagen fibers that are primarily oriented in the horizontal plane. A few fibroblasts and fibrocytes and a small amount of ground substance also are found in this layer (see Supporting Cells and Extracellular Matrix of Connective Tissue: Functional Components). The collagen fibers of the capsule attach to the adjacent surfaces of the superior and inferior articular processes, just peripheral to the articular cartilage. In fact, a gradual transition occurs from the joint capsule to fibrocartilage and finally to the articular cartilage of the Z joint. The capsules have a rich sensory innervation, consisting of mechanoreceptors for proprioception and free nerve endings containing substance P for nociception (Giles & Taylor, 1987; Yamashita et al., 1996). However, they have a poor blood supply, which slows the healing of these structures once they are damaged (Giles, 1992b). The multifidus lumborum muscle attaches to the articular capsule, which lies just medial to the primary attachment of this muscle to the mamillary process. The multifidus lumborum muscle may put tension on the capsule and help keep it from being entrapped in the joint space (Taylor & Twomey, 1986).
The posterior and lateral aspect of each lumbar inferior articular process (IAP) has a “lip” that projects further posteriorly than the medial aspect of the IAP, which is more anteriorly located. Consequently, the articular capsule “wraps around” this posterior lip of the lateral aspect of the IAP before attaching to the more anteriorly positioned medial aspect of the IAP. The cervical and thoracic IAPs are oriented differently and do not have this posterior lip; consequently, their capsules do not have a wrap-around component. Boszczyk and colleagues (2001) found that this wrap-around region of the lumbar Z joint capsule was thicker and more fibrocartilaginous in nature (containing type II collagen, aggrecan, and link protein) than the thoracic Z joint capsules, which were found to be thinner and more purely fibrous (rather than fibrocartilaginous) in nature. The entheses (attachment sites) of the lumbar Z joint capsules to the lumbar inferior and superior articular processes were found to have the same fibrocartilaginous composition as the wrap-around portion, indicating that traction forces were placed on the entheses. The authors believed that the costovertebral (costocorporeal) and costotransverse articulations of the thoracic region, along with the spatial orientation of the thoracic articular processes, spared the thoracic capsules from the traction and compressive forces placed on the lumbar Z joint capsules (Boszczyk et al., 2001).
A detailed description of the fiber direction of the outer part of the lumbar Z joint capsules and the clinical significance of the fiber direction in the lumbar capsule is given in Chapter 7.
The articular capsules are thinner superiorly and inferiorly, where they form capsular recesses that cover fat-filled synovial pads. Defects exist within the superior and inferior aspects of the joint capsule and allow for the passage of small nerves and vessels. The synovial joint recesses and the development of synovial joint cysts are discussed in further detail with the lumbar region, where they have been studied the most extensively (see Chapter 7). Also, the specific innervation of the Z joint capsule by the medial branch of the posterior primary division (dorsal ramus) is discussed in Chapter 2.
Ligamentum Flavum
The ligamentum flavum takes the place of the joint capsule anteriorly and medially. As discussed, this ligament passes from the anterior and inferior aspect of the lamina of the vertebra above to the posterior and superior aspect of the lamina of the vertebra below. However, the lateral fibers of this ligament course anterior to the Z joint, attach to its margins, and form its anterior capsule. Synovial extensions, or cysts, protrude out of the Z joint and along the attachment sites of the ligamentum flavum to the adjacent superior and inferior articular processes.
However, as described in Chapter 7, many instances of ligamenta flava hypertropy are probably the result of inflammation related to repeated microtears in the ligament. The inflammation then leads to hypertrophic scar formation (fibrosis) (Sairyo et al., 2007).
The ligamentum flavum can also ossify over a long period of time, which can lead to serious vertebral canal stenosis. Ossification of the ligamentum flavum has a higher prevalence in the Asian population than in other racial groups. The ossification process appears to be related to degeneration of the elastic fibers, which in the case of ossification of the ligamentum flavum appears to have a genetic component (Yayama et al., 2007).
Synovial Membrane
The synovial membrane, synovium, or joint lining is a condensation of connective tissue that covers the inner surface of the fibrous capsule, thus forming a sac that encloses the joint cavity (Fig. 14-7). Therefore the region of a diarthrodial joint surrounded by a synovium is known as the synovial, or joint, cavity. The synovium covers the nonarticular bone enclosed within the joint capsule and courses to the margin of the articular cartilage, where a transition zone exists between the synovium and articular cartilage. The synovium does not cover the load-bearing surface of the cartilage. The joint cavity normally contains a small amount of a highly viscous, hyaluronic acid–rich fluid that lubricates the joint surfaces. This fluid is known as synovial fluid and is produced by the cells within the synovial membrane (see Synoviocytes). The major function of the synovial membrane is to produce synovial fluid. Another function is to absorb waste products of metabolism and cellular debris before they can accumulate in the Z joint cavity.
FIG. 14-7 Portion of the zygapophysial (Z) joint at a magnification of approximately ×40. The region here is shown by the box in Figure 14-1. Portions of the articular cartilage, subchondral bone of the superior articular process and mamillary process, the articular capsule, and the Z joint synovial fold can be seen.
Typically projections of the synovial layer extend into the synovial cavity as Z joint synovial folds (Giles, 1992a). Their purpose is to fill in the small gaps along the periphery of the joint, where the articular cartilages of the opposing surfaces do not normally come in contact with one another. These folds also produce synovial fluid and provide an efficient mechanism for the distribution of this fluid directly into the joint cavity.
Z joint synovial folds contain a relatively large amount of adipose tissue at the region of their attachment to the fibrous layer of the articular capsule. They possess a nociceptive sensory nerve supply of free nerve endings containing substance P (Giles, 1987), and at times they may extend a considerable distance into the joint, in which case their central tips usually are fibrous. Entrapment of these folds between and extrapment of them peripheral to the articular surfaces of the Z joint have been implicated as possible causes of back pain (Mooney & Robertson, 1976; Giles & Taylor, 1987; Bogduk, 2005). Giles (1992a) also states that traumatic synovitis of these folds may cause the release of pain-mediating agents and subsequent back pain.
Synoviocytes
Transmission electron microscopy studies reveal that a discontinuous layer of cells, known as synoviocytes, lines the free surface of the synovial membrane. Although synoviocytes resemble other connective tissue cells, they differ from ordinary fibroblasts (see Table 14-1) in their ultrastructural features and metabolic activities.
The synovial fluid produced by the type A synoviocytes is rich in hyaluronic acid and also contains protein, although its protein content is less than that of blood plasma (Triano, 1992; Standring et al., 2008). The hyaluronic acid imparts synovial fluid with great viscosity. Coiling of the hyaluronic acid molecules and interlocking between different molecules allow the synovial fluid to act as a shock absorber during compressive loads. However, during shear forces the coiled hyaluronic acid molecules straighten and the interlocking between molecules decreases, resulting in smooth, low-friction movement between the adjacent Z joint surfaces.
Supporting Cells and Extracellular Matrix of Connective Tissue: Functional Components
Mature Connective Tissue
Cells of connective tissue: As mentioned, connective tissue consists of cells, fibers, and ground substance (including water). The type of supportive resident cells found in connective tissue varies considerably and may include fibroblasts; chondroblasts and chondrocytes; and osteoblasts, osteoclasts, and osteocytes. These cells are important when considering the connective tissue of spinal structures. Adipocytes, mast cells, macrophages, and myofibroblasts are also found in connective tissue in various parts of the body. The functions and primary characteristics of these cells are listed in Table 14-1.
In addition to the fixed or resident cells of connective tissue described in Table 14-1, connective tissue also contains transient or immigrant cells. These include all the formed cellular elements found in blood with the exception of erythrocytes. The immigrant cells include the neutrophils, eosinophils, basophils, monocytes, lymphocytes, and plasma cells. When inflammation occurs, these immigrant cells leave the circulation and join fibroblasts and other connective tissue resident cells, such as macrophages. Once in the connective tissue, they fight microorganisms that cause inflammation and clean up (phagocytize) the debris that results from this process.
Collagen Synthesis
It was believed for many years that collagen synthesis occurred primarily in fibroblasts, chondroblasts, osteoblasts, and odontoblasts; however, recent investigations in collagen biology indicate that many other cell types produce this unique protein. Collagen synthesis has been studied extensively in fibroblasts (Standring et al., 2008). Fibroblasts have the extensive rough endoplasmic reticulum and well-developed Golgi apparatus required of cells actively involved in protein synthesis. Labeled amino acids endocytosed by fibroblasts can be followed autoradiographically to the rough endoplasmic reticulum (rER), later to the Golgi complex, then to the outside of the fibroblast, and eventually to the newly formed collagen fibers. This evidence indicates that the collagen synthesis pathway is similar to that of other proteins. Fibroblasts synthesize collagen de novo and secrete it into the extracellular matrix. Fibroblasts also have the ability to break down collagen with specific degradative enzymes called collagenases.
Collagen synthesis begins inside cells. However, the final processing and assembly into fibers takes place after collagen building blocks have been secreted outside the manufacturing cells. The intracellular events include synthesis of proalpha chains in the rER, hydroxylation and glycosylation of proalpha chains into triple helices in the Golgi apparatus, and formation of secretory granules (vesicles). The extracellular events include cleavage of extension peptides, fibrillogenesis and cross-linking, and assembly of fibrils into mature fibers (Fig. 14-8). Box 14-1 shows the events (steps) involved in collagen synthesis within the fibroblast (steps 1 through 9) and outside the fibroblast in the extracellular matrix (steps 10 through 12).
The amino acid composition of collagen is one of the features that make collagen such a unique protein. Four amino acids compose most of the polypeptides in the collagen macromolecules. The principal amino acids that make up collagen are glycine (35%), proline (12%), hydroxyproline (10%), and alanine (11%). In the cytoplasm of the fibroblast, approximately 250 to 300 amino acids are combined by polyribosomes associated with rER to form a polypeptide with a molecular weight of 30,000 Da. This step of translation is performed under the control of messenger ribosomal ribonucleic acid (mRNA). Three polypeptide chains are combined into polypeptide alpha triple helices with a molecular weight of approximately 100,000 Da. These triple helices are released into the cisternae of rER (see Box 14-1, steps 1 through 3). Glycine is the third amino acid in each alpha chain of the newly formed triple helix. The amino acid after glycine frequently is proline, and the amino acid preceding the glycine frequently is hydroxyproline. Differences in the chemical structure of the alpha chains are responsible for at least 19 different types of collagen identified to date (Ross et al., 2003). Specifics of the 11 most common types of collagen can be found in Table 14-3.
Several modifications of the polypeptide chains occur within the cisternae of rER and the Golgi apparatus (see Box 14-1, steps 4 to 6, and Fig. 14-8). Disulfide bonds are formed within each polypeptide chain and between adjacent chains. Vitamin C is necessary for the formation of the disulfide bonds, and its absence results in certain types of collagen-related diseases such as scurvy. This bonding gives shape and stability to the triple-helix collagen macromolecule. The structure formed now constitutes a procollagen molecule. The procollagen molecule moves to the exterior of the cell via secretory granules (see Box 14-1, steps 7 to 9, and Fig. 14-8). Further modifications are made outside the cell. For example, enzymes cleave most of the uncoiled amino acids, thereby converting procollagen to tropocollagen molecules. These eventually aggregate to produce collagen fibrils (see Box 14-1, steps 10 to 12). Cross-links between lysine and hydroxylysine are then formed, giving the molecule its tensile strength. Changes in collagen cross-links have been seen in IVD degeneration (Duance et al., 1998).
The tropocollagen molecules are 300 nm long and 1.5 nm in diameter. They consist of three polypeptide chains that are twisted around one another to form a right-handed superhelix with a head and a tail end. Numerous tropocollagen molecules lie end-to-end and also in parallel chains or rows. All the molecules face the same direction, and approximately one fourth of the length of the tropocollagen molecule overlaps between the parallel rows. Therefore a tropocollagen molecule of one row ends approximately one fourth of the distance along the length of another tropocollagen molecule of an adjacent row. This configuration results in a regular 64- to 67-nm periodicity that is clearly visible on an electron micrograph. Figure 14-9 shows collagen fibers within the IVD.
FIG. 14-9 A, Sagittal section of two adjacent vertebrae and the intervertebral disc between them. B, Illustration of the boxed region in A at higher magnification (magnification ≈×15). In addition to showing the anulus fibrosus (AF), nucleus pulposus, and cartilaginous end plate, the vertebral body and posterior longitudinal ligament are shown. Notice that the outer fibers of the AF attach to the cortical and subchondral bone of the vertebral body. These attachment sites are known as Sharpey’s fibers. The collagen fibers of the inner layers of the AF enter the end plate and curve to run parallel to the discal surface of the vertebral body. (The boxes labeled A, B, and C refer to the regions shown in Figure 14-10.)
The finest strand of collagen that can be seen with the light microscope is the fibril, which is approximately 0.2 to 0.3 µm in diameter. A fibril is made up of still smaller units that have a diameter of 45 to 100 nm. These are called microfibrils. Newly formed microfibrils are only approximately 20 nm in diameter, and evidence shows that they increase in size with age. Most microfibrils are visible only with the electron microscope and demonstrate the characteristic cross-banding with a periodicity of 64 to 67 nm. The parallel assembly of microfibrils forms fibrils. The fibrils in turn aggregate in bundles to form the thicker collagen fibers. These fibers have a diameter ranging from 1 to 12 µm or more.
Types of Collagen
At present, 19 different types of collagen have been positively identified. They are designated as types I through XIX. Types I to V are the most abundant types of collagen. Types VI to XIX are considered less important because they occur in small quantities. Several of the minor types of collagen (types VI, IX, X, XI, XII, and XIV) are present in small amounts in the IVD (Duance et al., 1998). Table 14-3 lists the characteristics of the 11 most important types of collagen.
Types I, II, and III are arranged as ropelike fibrils and are the main forms of fibrillar collagen. Type I collagen consists of two alpha-1 chains and one alpha-2 chain and represents 90% of all collagen fibers distributed in connective tissue. Because type I fibers resist tensile stresses, their orientation and cross-linking vary according to the local environment. Type I collagen is found in bone, tendon, and the anulus fibrosus (AF) of the IVD. It is also found in the skin and cornea (see Table 14-3).
Type II collagen fibers are small, banded fibrils averaging 20 nm in diameter. They help to form the extracellular matrix of hyaline cartilage, including that of the Z joints and cartilaginous end plates (CEPs) of the IVDs. Type II collagen is the main type of collagen found in the nucleus pulposus (NP) of the IVD. It is also found in elastic cartilage and the cornea and vitreous body of the eye. These fibers demonstrate a high electrostatic attraction for the chondroitin sulfate glycosaminoglycans. Type II collagen contains a higher degree of lysine hydroxylation than type I collagen.
Types III and IV collagen are well distributed throughout the body but are not found to any great extent in Z joints, IVDs, or other spinal tissues, although type III has been found in regions adjacent to spondylosis (Schollmeier, Lahr-Eigen, & Lewandrowski, 2000). The key features of these fibers and collagen types V through XI are listed in Table 14-3.
Ground Substance
The cells and fibers of connective tissue are surrounded by a translucent, fluidic, homogeneous, gel-like matrix called amorphous ground substance (Bloom & Fawcett, 1986). The ground substance exhibits no structural organization that is visible with light microscopy. Extracellular amorphous ground substance plays a vital role in the regulation of tissue nutrition, support, and maintenance of proper water content. Based on chemical analysis, the extracellular ground substance of connective tissue has the physical properties of a viscous solution or thin gel and consists of proteoglycans and glycosaminoglycans of various types. Proteoglycans and glycosaminoglycans are an important part of the hyaline cartilage of the Z joints and the cartilaginous (vertebral) end plates of the IVDs. They are also being studied with regard to the AF and NP of the IVD. Therefore glycosaminoglycans and proteoglycans are discussed in further detail with the articular cartilage of the Z joint (see previous discussion) and with the IVD (see following discussion).
Microscopic and Molecular Structure of the Intervertebral Discs
Symphyseal joints unite the vertebral bodies, and these joints are made up of the IVDs. The IVDs permit a limited amount of movement between the vertebral bodies while maintaining a union of great strength. The intrinsic stability of the motion segment (two adjacent vertebrae and the ligaments, including the disc, between them), and therefore of the whole spine, results mainly from the IVDs and the ligaments associated with them (Bogduk, 2005). The paraspinal and trunk muscles provide the spine’s extrinsic stability.
IVDs (see Fig. 14-9) are important parts of the spinal column and play an active and important role in the spine’s physiologic function. The physical properties, elasticity, and resiliency of the IVDs allow them to give support to the spine and allow motion to occur between adjacent vertebral segments, while also preventing too much motion from occurring between the same segments. The IVDs also allow the spine to return to its original shape after being compressed or stretched (Chai & Tang, 1987).
The IVD consists of three main parts: the outer AF, which consists of a series of fibrocartilaginous rings (except in the cervical region, where it is a solid, crescent-shaped, fibrocartilaginous structure); the inner gelatinous NP; and the CEPs of hyaline-like cartilage. The end plates are located between the bony vertebrae and other parts of the IVD (Ghosh, 1990).
Each IVD is reinforced peripherally by circumferential ligaments (see Fig. 14-9, A). A thick anterior longitudinal ligament extends down the anterior aspect of the spinal column and is attached to the vertebral end plates. It provides additional anterior support to the AF. A thinner posterior longitudinal ligament spans across the posterior aspect of each disc and is firmly attached to the IVD’s posterior aspect.
The IVD is specialized connective tissue designed to provide strength, mobility, and resistance to strain. All three parts of the IVD listed previously (NP, AF, and CEP, Fig. 14-10; see also Fig. 14-9, B) consist of water, cells, proteoglycans (PGs), and collagen. These components are found in varied concentrations in the three different regions of the disc. In fact, the varied concentrations of these basic components within the IVD make it a specialized type of connective tissue. For example, after an early age (≈2 years) the disc has no blood supply (except for the vessels within the vertebral bodies that are adjacent to the CEPs and remain until 11 to 12 years of age), and so receives its nutrition from the adjacent vertebral bodies. The PGs are essential in the process of attracting fluid and nutrition to the IVD from the adjacent vertebral bodies. The PGs are negatively charged and attract Na+, which then attracts water and other nutrients by osmotic flow. The PGs have been found to actually regulate the amount and type of molecules entering the IVD. Breakdown of PG molecules in the IVD has been associated with the decreased fluid and increased tissue breakdown found in disc degeneration. PGs are important to the health and treatment of the IVD. Because chondroitin sulfate is a major component of the PGs, this substance and a related molecule, glucosamine sulfate, are frequently given as part of the conservative treatment of disc degeneration.
FIG. 14-10 Regions of the intervertebral disc. A, B, and C correspond to the respective lettered boxes of Figure 14-9, BA, Cartilaginous end plate. B, Nucleus pulposus. C, Anulus fibrosus. (A, B, and C represent a magnification ≈×100.)
Collagen is another of the important components of the IVDs. As mentioned, the main type of collagen in the AF is type I, and is a ropelike molecule that is tough and strong and gives the AF its ability to withstand the forces and loads placed on the IVD. The NP has a higher concentration of the less–well-organized type II collagen.
Therefore each of the building blocks of the IVD is important and clinically relevant. In addition, each of the four constituents is closely related to the others. For example, the collagen fibers within the IVD become taut during movements of the spine and tend to restrain the PGs. The PGs in turn allow the IVD to deform. Because of its ability to absorb fluid (swell) and then to maintain its hydration (water), the PG gel of the NP is able to resist compression under large external loads (Weiss, 1988). The cells in turn maintain the proper levels of PGs and collagen fibers. Therefore the IVD is able to act as a relatively thick lubricating pad that prevents adjacent vertebrae from being eroded by abrasive forces during movement of the spinal column. The hydrated gelatinous NP serves to a certain extent as a shock absorber to reduce the impact between adjoining vertebrae (Mescher, 2010), although the vertebral body is primarily responsible for this function.
The histologic changes that take place in the IVD with advancing age have been described in postmortem studies by several investigators (Brown, 1971; Pritzker, 1977; Roberts et al., 1989, 1996; Boos et al., 2002). These changes include loss of distinction between the NP and AF, desiccation and fibrosis of the NP with fibrillation of the matrix, brown discoloration of the nucleus, fissuring of the nucleus and AF, fractures of the vertebral end plate, and formation of osteophytes.
Figure 14-11 demonstrates a series of events associated with degeneration of the IVD. Based on plain x-ray films, the fundamental diagnostic features of disc degeneration are reported to be disc space narrowing and osteophytosis. Decreased hydration as demonstrated by a decreased signal intensity of the IVD on T1- and T2-weighted magnetic resonance imaging (MRI) scans is also an indication of IVD degeneration. The section entitled Normal Aging of the Intervertebral Discs and Intervertebral Disc Degeneration discusses the changes of IVD degeneration in further detail. In addition, Chapter 7 describes the consequences of these changes and the development of internal disc disruption. Chapter 2 describes the gross anatomic features of the IVD and the clinical relevance of these features, and Chapter 11 discusses IVD bulging, protrusion, and extrusion and their effects on the neural elements within the vertebral canal (i.e., cauda equina and dorsal root ganglia).
FIG. 14-11 Flowchart demonstrating a series of events leading to degeneration of the intervertebral disc.
Anulus Fibrosus
The AF is the rigid, outer series of rings (lamellae) that forms the peripheral portion of the IVD (Figs. 14-12 and 14-13). It functions to absorb pressure from the central well-hydrated (jellylike) NP. The tightly packed collagen fibers of the AF normally do not allow the large PG molecules of the NP to pass between them, even when the IVD is subjected to large compressive forces. The adult AF is not distinctly separated from the NP or cartilage of the vertebral end plates (Inerot & Axelsson, 1991).
FIG. 14-13 Midsagittal section through a cadaveric lumbar spine. Notice that the cartilaginous end plates, the anuli fibrosi, and the nuclei pulposi can be seen at several levels. Also notice the Schmorl’s node (intravertebral herniation), which has been labeled. (Compare with Figs. 14-15 and 14-16.)
The outer ring of the AF consists of an external tough layer of dense collagenous connective tissue, whereas the remainder of the AF is primarily composed of overlapping concentric layers of fibrocartilage. The outer part of the AF attaches to the margins of adjacent CEPs in infancy and childhood and to the outer rims of adjacent vertebral bodies (region of the anular apophyses) in adolescence (see Fig. 14-9, B, Sharpey’s fibers). The attachments of the AF to the anular (ring) apophyses are considered to be a part of the intervertebral disc (Fardon, 2001).
Light and electron microscopy indicate that a typical lumbar AF is composed of fibrocartilage and has a lamellar structure. Anteriorly the AF consists of more than 20 moderately thick lamellae. The outer lamellae are entirely fibrous and contain thick, tightly packed bundles of type I collagen fibers (Ghosh, 1990; Schollmeier, Lahr-Eigen, & Lewandrowski, 2000). Although the outer AF is composed of type I collagen (see Table 14-3), the fibers of the inner AF are composed of type II collagen (Bishop, 1992; Schollmeier, Lahr-Eigen, & Lewandrowski, 2000). The lamellae of the inner part of the AF also have a richer PG ground substance associated with them (greater concentration of PGs in the posterior versus the anterior AF) (Iatridis et al., 2007), which increases the capacity to resist compression (McDevitt, 1988). The collagen fibers in each lamella are orientated parallel to one another and form an angle of inclination (≈25 to 30 degrees) with the horizontal axis of the bony vertebral rims. The fibers of each consecutive layer form approximately a 120- to 130-degree angle with the fibers of adjacent lamellae. The lamellar structure and the angle of inclination of the collagen fibers enable the AF to sustain the normal forces of compression, torsion, and flexion that occur during movements of the IVD (Chai & Tang, 1987). Elastic fibers have also been identified in the outer and inner aspects of the AF and may also play a role in the mechanical properties of the IVD (Yu et al., 2005).
As mentioned, the anterior and lateral parts of the AF are composed of more than 20 moderately thick lamellae. The outer lamellae are loosely attached to the strong anterior longitudinal ligament (Ghosh, 1990). The posterior and posterolateral parts of the AF are much thinner. They consist of 12 to 15 more closely arranged, thinner lamellae that follow the contour of the posterior parts of the adjacent vertebral bodies. The collagen fibers of the outer lamellae of the AF are fused with the lateral margin of the relatively thin posterior longitudinal ligament (Ghosh, 1990). As mentioned, the outer collagen fibers also attach to the posterior vertebral rims. The inner fibers of the AF are continuous with the CEPs (see following discussion and Fig. 14-9, B).
The cells of the AF are primarily chondrocytes. Although they produce cartilage, specific matrix proteins, the chondrocytes of the AF are of a different stage of differentiation than the chondrocytes of the growth plates of bones or of articular cartilage (Poiraudeau et al., 1999).
PG extraction from ground human lumbar AFs suggests that the PGs contain three regions: a chondroitin sulfate–rich region, a keratan sulfate–rich region, and a region that binds to hyaluronic acid (Table 14-4; see also Fig. 14-6). By binding to hyaluronic acid, the PGs are permitted to aggregate into PG macromolecules. Because of the immense clinical importance of PGs as they relate to the IVDs, a section devoted to this topic is found later in this chapter. However, characteristics of PGs specific to the AF are covered here.
Table 14-4
∗Five main groups of glycosaminoglycans with different tissue distributions exist. Chondroitin sulfate exists as chondroitin 4-sulfate and chondroitin 6-sulfate; both possess high levels of interaction with collagen type II. Dermatan sulfate demonstrates low levels of interaction, mainly with collagen type I. Heparan sulfate demonstrates intermediate levels of interaction with collagen types III and IV. Sulfation causes the molecules to be highly negatively charged and contributes to their ability to attract and bind Na+ and water.
Previous investigations of glycosaminoglycans and PGs of the IVDs have found that the PGs from the AF contain approximately 75% chondroitin sulfate and 25% keratan sulfate and hyaluronic acid (Antonopoulos et al., 1974; Stevens, Dondi, & Muir, 1979). These percentages are determined by analyzing the glucosamine/galactosamine ratios (see Table 14-4) (Inerot & Axelsson, 1991). Both the hyaluronic acid and the keratan sulfate concentrations are higher in the AF than in hyaline cartilage (Antonopoulos et al., 1964; Hardingham & Adams, 1976). Also, the keratan sulfate region appears to be larger in AF PGs than in hyaline cartilage PGs. Fibrocartilage of human knee joint menisci has been shown also to contain dermatan sulfate. This molecule has not been detected in the human AF. Biochemically the absence of dermatan sulfate and the presence of types I and II collagen fibers suggest that the AF may be classified as an intermediate between hyaline cartilage and fibrocartilage (Inerot & Axelsson, 1991).
A study of the aging of IVD PG composition of canines and humans has shown that the keratan sulfate–rich region of the PG core protein (Fig. 14-6) is more resistant to proteolysis than the chondroitin sulfate–rich region. In addition, the number of keratan sulfate–rich fragments in human disc tissue increases with aging (Cole, Ghosh, & Taylor, 1986).
Clinical Considerations
Sudden movements of the lumbar spine, especially torsion coupled with flexion, can produce small tears in the AF. These tears usually occur in the posterior part of the AF, where the distribution of collagen fibers is less concentrated. Sometimes, tears in the AF may allow some of the soft, jellylike NP to squeeze out into the vertebral canal. This latter condition is known as an extruded IVD (see Chapter 11). IVD extrusion is not as common a cause of back pain as once thought (see Chapter 7). However, the discs can be a source of pain without protrusion or extrusion (Bogduk, 1990). Contrary to previous reports (Malinsky, 1959; Wyke, 1987) that the IVD could not produce pain because it lacks nerve supply, several investigators (Yoshizawa, O’Brien, & Thomas-Smith, 1980; Bogduk et al., 1981) have confirmed that the lumbar discs do have a nerve supply and that nerve fibers and nerve endings have been demonstrated to exist in at least the outer third and possibly as far as the outer half of the AF. Most of these authors conclude that the lumbar disc is supplied with the necessary apparatus for the transmission of nociception, resulting in the subsequent perception of pain. Chapters 2, 7, and 11 discuss the gross anatomy, including the innervation, and the clinical relevance of the IVD (including the AF) in further detail.
Nucleus Pulposus
Both fetal and infant discs have large notochordal NPs with abundant fluid mucoid matrices. The nucleus of a young disc is encapsulated along the periphery by the AF and on the superior and inferior surfaces by the CEPs (see following discussion). Perinatally the AF and CEPs are vascular, but their blood supply declines dramatically with childhood growth (Taylor, 1990); by 11 to 12 years of age, even the blood vessels that earlier supplied the IVD by entering the deepest parts of the CEPs from the vertebral bodies cannot be found. In fact, the adult IVD (including the NP) is the largest avascular structure of the body. It receives nutrition primarily by means of diffusion from blood vessels within the subchondral bone of the adjacent vertebral bodies. This diffusion process by which the IVD receives its nutrients is known as imbibition.
The human NP is a highly hydrated tissue at birth, with a water content of 88%. This falls to 69% at 77 years of age. By comparison, the water content of the AF declines from 78% at birth to approximately 70% at 30 years, and thereafter it stays relatively constant (Gower & Pedrini, 1969). In adults, as the hydration declines with age, the tissues become firmer and lose their translucency, and the boundaries between the NP and AF become less distinguishable. Table 2-5 shows the relative concentrations of water, collagen, and PG (nonaggregated/aggregated ratio) of the NP and AF.
The higher water content of the NP, compared with that of the AF, is accompanied by a lower concentration of collagen in the NP. In addition, the collagen found in the NP is type II rather than type I, which is found in the AF. The individual fibrils of type II collagen are much smaller than those of type I (see Table 14-3). The fibers are also loosely arranged and are surrounded by a more abundant ground substance. In the NP, this ground substance contains a high percentage (65%) of hydrophilic, nonaggregated PGs (Iatridis et al., 2007).
Therefore the NP is a thick, jellylike region with a high concentration of fluid. It draws this fluid from the surrounding vertebral bodies. The fluid, a distillate of plasma, passes through the CEPs on its way to the NP. The NP also has relatively few cells. The cells are primarily notochordal cells in the young (see following discussion). These are then replaced by fibroblasts and chondrocytes. The adult NP comprises 35% to 50% of the IVD (Bishop, 1992). It normally lies slightly posterior to the IVD’s center. Normal nuclear material moves backward and forward with flexion and extension movements of the spine, respectively.
The region of the adult NP that is adjacent to the CEPs contains a relative abundance of chondrocytes. The matrix surrounding the chondrocytes stains deeply with safranin and Alcian blue because of the presence of abundant PG macromolecules. Also in this region, vertically oriented collagen fibers extend from the end plate to the NP (Oda, Tamaka, & Tsukuki, 1988
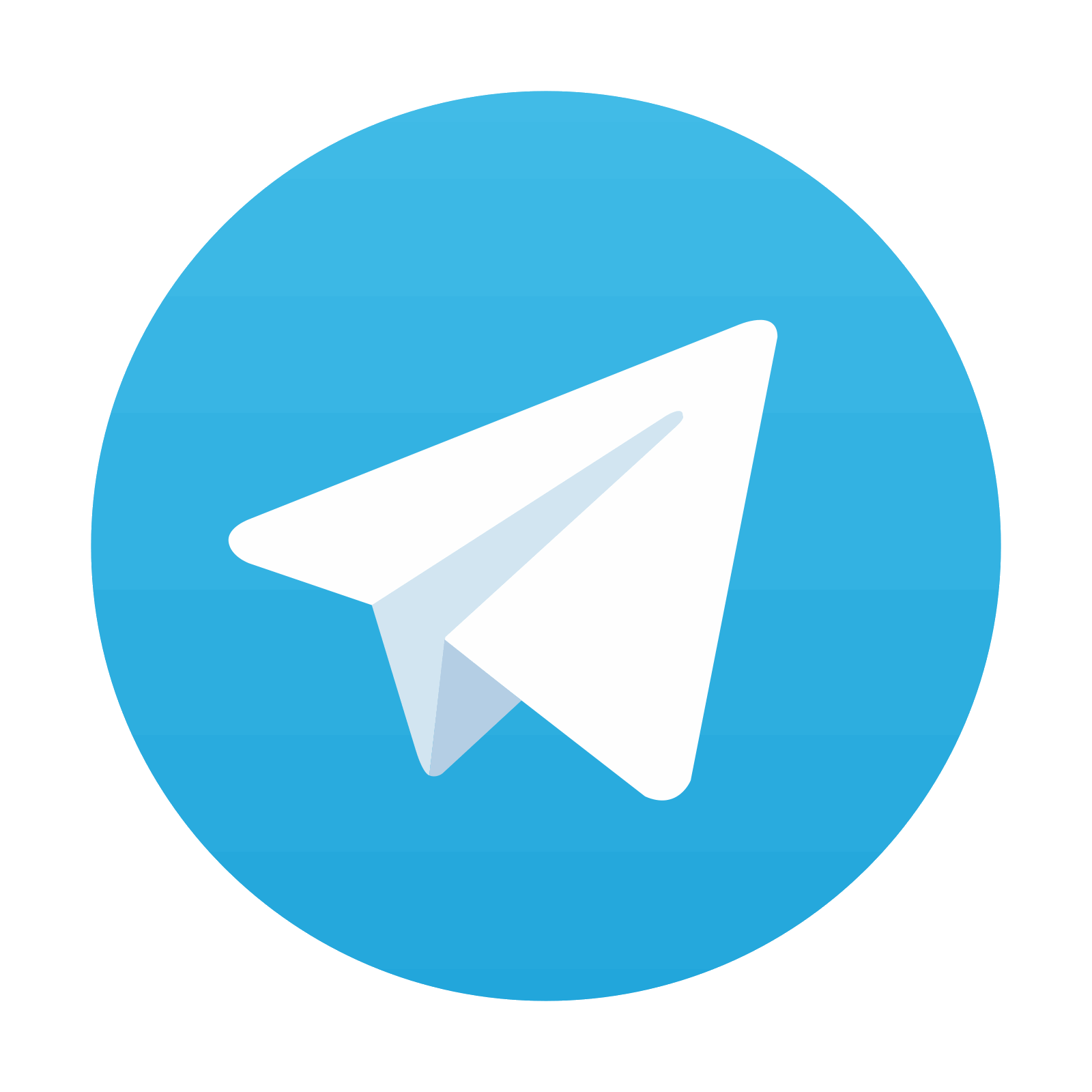
Stay updated, free articles. Join our Telegram channel
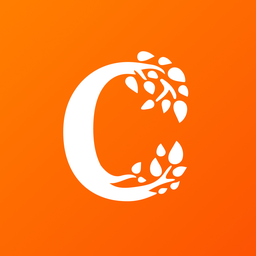
Full access? Get Clinical Tree
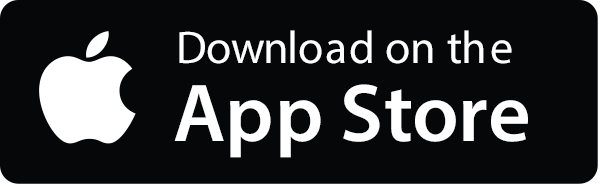
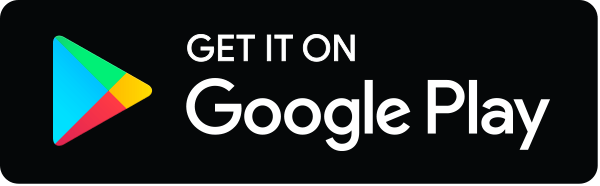