ROLE OF METABOLISM IN BIOSYNTHESIS AND GROWTH
Microbial growth requires the polymerization of biochemical building blocks into proteins, nucleic acids, polysaccharides, and lipids. The building blocks must come preformed in the growth medium or must be synthesized by the growing cells. Additional biosynthetic demands are placed by the requirement for coenzymes that participate in enzymatic catalysis. Biosynthetic polymerization reactions demand the transfer of anhydride bonds from adenosine triphosphate (ATP). Growth demands a source of metabolic energy for the synthesis of anhydride bonds and for the maintenance of transmembrane gradients of ions and metabolites.
Metabolism has two components, catabolism and anabolism (Figure 6-1). Catabolism encompasses processes that harvest energy released from the breakdown of compounds (eg, glucose), and using that energy to synthesize ATP. In contrast, anabolism, or biosynthesis, includes processes that utilize the energy stored in ATP to synthesize and assemble the subunits, or building blocks, of macromolecules that make up the cell. The sequence of building blocks within a macromolecule is determined in one of two ways. In nucleic acids and proteins, it is template-directed: DNA serves as the template for its own synthesis and for the synthesis of the various types of RNA; messenger RNA serves as the template for the synthesis of proteins. In carbohydrates and lipids, on the other hand, the arrangement of building blocks is determined entirely by enzyme specificities. Once the macromolecules have been synthesized, they self-assemble to form the supramolecular structures of the cell, eg, ribosomes, membranes, cell wall, flagella, and pili.
FIGURE 6-1
The relationship between catabolism and anabolism. Catabolism encompasses processes that harvest energy released during disassembly of compounds, using it to synthesize adenosine triphosphate (ATP); it also provides precursor metabolites used in biosynthesis. Anabolism, or biosynthesis, includes processes that utilize ATP and precursor metabolites to synthesize and assemble subunits of macromolecules that make up the cell. (Reproduced with permission from Nester EW, Anderson DG, Roberts CE, Nester MT [editors]: Microbiology: A Human Perspective, 6th ed. McGraw-Hill, 2009, p. 127. © The McGraw-Hill Companies, Inc.)
The rate of macromolecular synthesis and the activity of metabolic pathways must be regulated so that biosynthesis is balanced. All of the components required for macromolecular synthesis must be present for orderly growth, and control must be exerted so that the resources of the cell are not expended on products that do not contribute to growth or survival.
This chapter contains a review of microbial metabolism and its regulation. Microorganisms represent extremes of evolutionary divergence, and a vast array of metabolic pathways is found within the group. For example, any of more than half a dozen different metabolic pathways may be used for assimilation of a relatively simple compound, benzoate, and a single pathway for benzoate assimilation may be regulated by any of more than half a dozen control mechanisms. Our goal is to illustrate the principles that underlie metabolic pathways and their regulation. The primary principle that determines metabolic pathways is that they are achieved by organizing relatively few biochemical-type reactions in a specific order. Many biosynthetic pathways can be deduced by examining the chemical structures of the starting material, the end product, and perhaps one or two metabolic intermediates. The primary principle underlying metabolic regulation is that enzymes tend to be called into play only when their catalytic activity is required. The activity of an enzyme may be changed by varying either the amount of enzyme or the amount of substrate. In some cases, the activity of enzymes may be altered by the binding of specific effectors, metabolites that modulate enzyme activity.
FOCAL METABOLITES AND THEIR INTERCONVERSION
The biosynthetic origins of building blocks and coenzymes can be traced to relatively few precursors, called focal metabolites. Figures 6-2, 6-3, 6-4, and 6-5 illustrate how the respective focal metabolites glucose 6-phosphate (G6PD), phosphoenolpyruvate, oxaloacetate, and α-ketoglutarate give rise to most biosynthetic end products.
Figure 6-2 illustrates how G6PD is converted to a range of biosynthetic end products via phosphate esters of carbohydrates with different chain lengths. Carbohydrates possess the empirical formula (CH2O)n, and the primary objective of carbohydrate metabolism is to change n, the length of the carbon chain. Mechanisms by which the chain lengths of carbohydrate phosphates are interconverted are summarized in Figure 6-6. In one case, oxidative reactions are used to remove a single carbon from G6PD, producing the pentose derivative ribulose 5-phosphate. Isomerase and epimerase reactions interconvert the most common biochemical forms of the pentoses: ribulose 5-phosphate, ribose 5-phosphate, and xylulose 5-phosphate. Transketolases transfer a two-carbon fragment from a donor to an acceptor molecule. These reactions allow pentoses to form or to be formed from carbohydrates of varying chain lengths. As shown in Figure 6-6, two pentose 5-phosphates (n = 5) are interconvertible with triose 3-phosphate (n = 3) and heptose 7-phosphate (n = 7); pentose 5-phosphate (n = 5) and tetrose 4-phosphate (n = 4) are interconvertible with triose 3-phosphate (n = 3) and hexose 6-phosphate (n = 6).
The six-carbon hexose chain of fructose 6-phosphate can be converted to two three-carbon triose derivatives by the consecutive action of a kinase and an aldolase on fructose 6-phosphate. Alternatively, aldolases, acting in conjunction with phosphatases, can be used to lengthen carbohydrate molecules: Triose phosphates give rise to fructose 6-phosphate; a triose phosphate and tetrose 4-phosphate form heptose 7-phosphate. The final form of carbohydrate chain length interconversion is the transaldolase reaction, which interconverts heptose 7-phosphate and triose 3-phosphate with tetrose 4-phosphate and hexose 6-phosphate.
The coordination of different carbohydrate rearrangement reactions to achieve an overall metabolic goal is illustrated by the hexose monophosphate shunt (Figure 6-7). This metabolic cycle is used by Cyanobacteria for the reduction of NAD+ (nicotinamide adenine dinucleotide) to NADH (reduced nicotinamide adenine dinucleotide), which serves as a reductant for respiration in the dark. Many organisms use the hexose monophosphate shunt to reduce NADP+ (nicotinamide adenine dinucleotide phosphate) to NADPH (reduced nicotinamide adenine dinucleotide phosphate), which is used for biosynthetic reduction reactions. The first steps in the hexose monophosphate shunt are the oxidative reactions that shorten six hexose 6-phosphates (abbreviated as six C6 in Figure 6-7) to six pentose 5-phosphates (abbreviated six C5). Carbohydrate rearrangement reactions convert the six C5 molecules to five C6 molecules so that the oxidative cycle may continue.
FIGURE 6-7
The hexose monophosphate shunt. Oxidative reactions (see Figure 6-6) reduce NAD+ (nicotinamide adenine dinucleotide phosphate) and produce CO2, resulting in the shortening of the six hexose phosphates (abbreviated C6) to six pentose phosphates (abbreviated C5). Carbohydrate rearrangements (see Figure 6-6) convert the pentose phosphates to hexose phosphates so that the oxidative cycle may continue.
Clearly, all reactions for interconversion of carbohydrate chain lengths are not called into play at the same time. Selection of specific sets of enzymes, essentially the determination of the metabolic pathway taken, is dictated by the source of carbon and the biosynthetic demands of the cell. For example, a cell given triose phosphate as a source of carbohydrate will use the aldolase–phosphatase combination to form fructose 6-phosphate; the kinase that acts on fructose 6-phosphate in its conversion to triose phosphate would not be expected to be active under these circumstances. If demands for pentose 5-phosphate are high, as in the case of photosynthetic carbon dioxide assimilation, transketolases that can give rise to pentose 5-phosphates are very active.
In sum, G6PD can be regarded as a focal metabolite because it serves both as a direct precursor for metabolic building blocks and as a source of carbohydrates of varying length that are used for biosynthetic purposes. G6PD itself may be generated from other phosphorylated carbohydrates by selection of pathways from a set of reactions for chain length interconversion. The reactions chosen are determined by the genetic potential of the cell, the primary carbon source, and the biosynthetic demands of the organism. Metabolic regulation is required to ensure that reactions that meet the requirements of the organism are selected.
Triose phosphates, formed by the interconversion of carbohydrate phosphoesters, are converted to phosphoenolpyruvate by the series of reactions shown in Figure 6-8. Oxidation of glyceraldehyde 3-phosphate by NAD+ is accompanied by the formation of the acid anhydride bond on the one carbon of 1,3-diphosphoglycerate. This phosphate anhydride is transferred in a substrate phosphorylation to adenosine diphosphate (ADP), yielding an energy-rich bond in ATP. Another energy-rich phosphate bond is formed by dehydration of 2-phosphoglycerate to phosphoenolpyruvate; via another substrate phosphorylation, phosphoenolpyruvate can donate the energy-rich bond to ADP, yielding ATP and pyruvate. Thus, two energy-rich bonds in ATP can be obtained by the metabolic conversion of triose phosphate to pyruvate. This is an oxidative process, and in the absence of an exogenous electron acceptor, the NADH generated by oxidation of glyceraldehyde 3-phosphate must be oxidized to NAD+ by pyruvate or by metabolites derived from pyruvate. The products formed as a result of this process vary and, as described later in this chapter, can be used in the identification of clinically significant bacteria.
FIGURE 6-8
Formation of phosphoenolpyruvate and pyruvate from triose phosphate. The figure draws attention to two sites of substrate phosphorylation and to the oxidative step that results in the reduction of NAD+ (nicotinamide adenine dinucleotide phosphate) to NADH (nicotinamide adenine dinucleotide hydride). Repetition of this energy-yielding pathway demands a mechanism for oxidizing NADH to NAD+. Fermentative organisms achieve this goal by using pyruvate or metabolites derived from pyruvate as oxidants.
Formation of phosphoenolpyruvate from pyruvate requires a substantial amount of metabolic energy, and two anhydride ATP bonds invariably are invested in the process. Some organisms—Escherichia coli, for example—directly phosphorylate pyruvate with ATP, yielding adenosine monophosphate (AMP) and inorganic phosphate (Pi). Other organisms use two metabolic steps: One ATP pyrophosphate bond is invested in the carboxylation of pyruvate to oxaloacetate, and a second pyrophosphate bond (often carried by guanosine triphosphate [GTP] rather than ATP) is used to generate phosphoenolpyruvate from oxaloacetate.
As already described, many organisms form oxaloacetate by the ATP-dependent carboxylation of pyruvate. Other organisms, such as E coli, which form phosphoenolpyruvate directly from pyruvate, synthesize oxaloacetate by carboxylation of phosphoenolpyruvate.
Succinyl-CoA is a required biosynthetic precursor for the synthesis of porphyrins and other essential compounds. Some organisms form succinyl-CoA by reduction of oxaloacetate via malate and fumarate. These reactions represent a reversal of the metabolic flow observed in the conventional tricarboxylic acid cycle (see Figure 6-11).
Conversion of pyruvate to α-ketoglutarate requires a metabolic pathway that diverges and then converges (Figure 6-9). In one branch, oxaloacetate is formed by carboxylation of pyruvate or phosphoenolpyruvate. In the other branch, pyruvate is oxidized to acetyl-CoA. It is noteworthy that, regardless of the enzymatic mechanism used for the formation of oxaloacetate, acetyl-CoA is required as a positive metabolic effector for this process. Thus, the synthesis of oxaloacetate is balanced with the production of acetyl-CoA. Condensation of oxaloacetate with acetyl-CoA yields citrate. Isomerization of the citrate molecule produces isocitrate, which is oxidatively decarboxylated to α-ketoglutarate.
ASSIMILATORY PATHWAYS
Acetate is metabolized via acetyl-CoA, and many organisms possess the ability to form acetyl-CoA (Figure 6-10). Acetyl-CoA is used in the biosynthesis of α-ketoglutarate, and in most respiratory organisms, the acetyl fragment in acetyl-CoA is oxidized completely to carbon dioxide via the tricarboxylic acid cycle (Figure 6-11). The ability to use acetate as a net source of carbon, however, is limited to relatively few microorganisms and plants. Net synthesis of biosynthetic precursors from acetate is achieved by coupling reactions of the tricarboxylic acid cycle with two additional reactions catalyzed by isocitrate lyase and malate synthase. As shown in Figure 6-12, these reactions allow the net oxidative conversion of two acetyl moieties from acetyl-CoA to one molecule of succinate. Succinate may be used for biosynthetic purposes after its conversion to oxaloacetate, α-ketoglutarate, phosphoenolpyruvate, or G6PD.
FIGURE 6-11
The tricarboxylic acid cycle. There are four oxidative steps, three giving rise to NADH (nicotinamide adenine dinucleotide hydride) and one giving rise to a reduced flavoprotein, Enz(FADH2). The cycle can continue only if electron acceptors are available to oxidize the NADH and reduced flavoprotein. GDP, guanosine diphosphate; GTP, guanosine triphosphate.
FIGURE 6-12
The glyoxylate cycle. Note that the reactions that convert malate to isocitrate are shared with the tricarboxylic acid cycle (see Figure 6-11). Metabolic divergence at the level of isocitrate and the action of two enzymes, isocitrate lyase and malate synthase, modify the tricarboxylic acid cycle so that it reductively converts two molecules of acetyl-CoA to succinate.
Similar to plants and algae, a number of microbial species can use carbon dioxide as a sole source of carbon. In almost all of these organisms, the primary route of carbon assimilation is via the Calvin cycle, in which carbon dioxide and ribulose diphosphate combine to form two molecules of 3-phosphoglycerate (Figure 6-13A). 3-Phosphoglycerate is phosphorylated to 1,3-diphosphoglycerate, and this compound is reduced to the triose derivative, glyceraldehyde 3-phosphate. Carbohydrate rearrangement reactions (see Figure 6-6) allow triose phosphate to be converted to the pentose derivative ribulose 5-phosphate, which is phosphorylated to regenerate the acceptor molecule, ribulose 1,5-diphosphate (Figure 6-13B). Additional reduced carbon, formed by the reductive assimilation of carbon dioxide, is converted to focal metabolites for biosynthetic pathways.
FIGURE 6-13
The Calvin cycle. A: Reductive assimilation of CO2. Adenosine triphosphate (ATP) and NADPH (nicotinamide adenine dinucleotide phosphate) are used to reductively convert pentose 5-phosphate (C5) to two molecules of triose phosphate (C3). B: The Calvin cycle is completed by carbohydrate rearrangement reactions (Figure 6-6) that allow the net synthesis of carbohydrate and the regeneration of pentose phosphate so that the cycle may continue. ADP, adenosine diphosphate.
Cells that can use carbon dioxide as a sole source of carbon are termed autotrophic
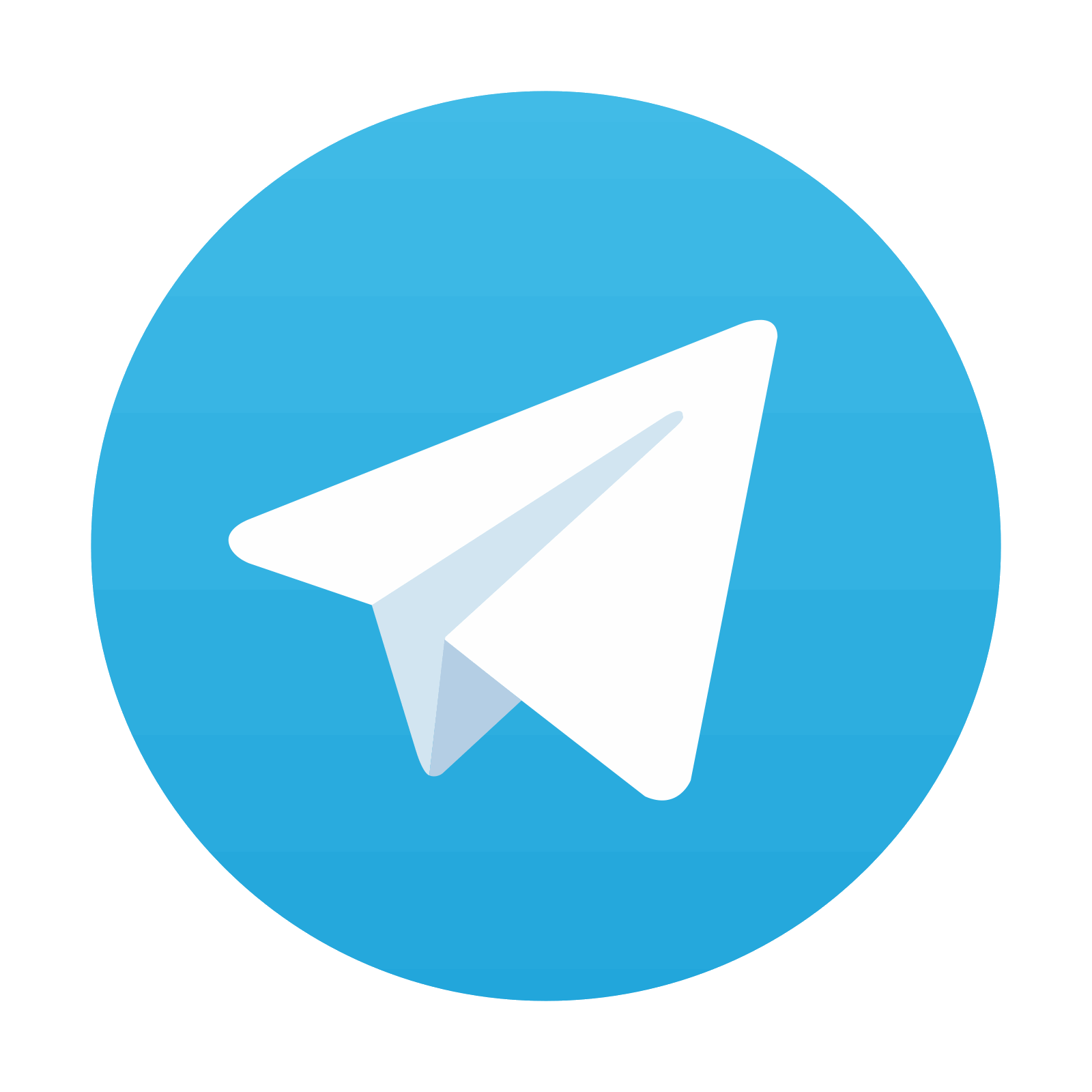
Stay updated, free articles. Join our Telegram channel
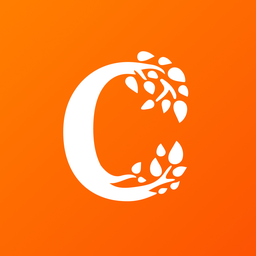
Full access? Get Clinical Tree
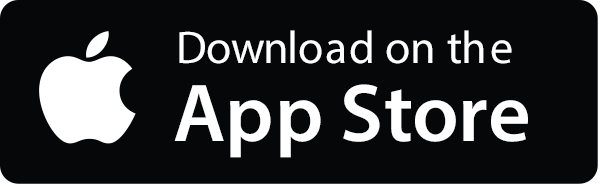
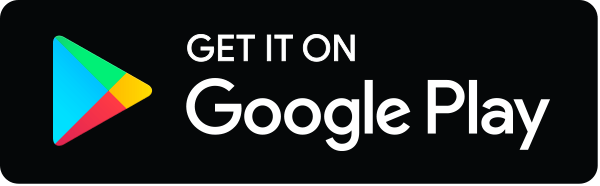
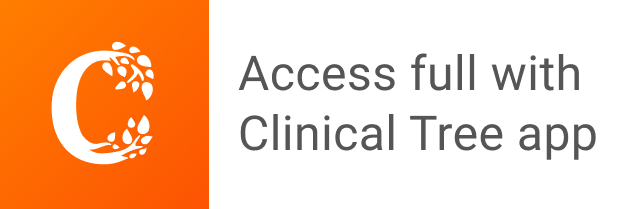