Chapter 18 Basic metabolic pathways and the origin of secondary metabolites
Although, because of varied form and function, it is not possible to illustrate a ‘typical’ plant cell Fig. 18.1A shows diagrammatically the structures that might be expected in an unspecialized young root cell. Such a cell possesses a rigid wall, which immediately distinguishes it from an animal cell, but no chloroplasts and only small vacuoles are present. In a green plant cell (Fig. 18.1B) the same components are present but the large vacuole has oppressed the nucleus and cytoplasm towards the wall; green plastids often with starch granules are common.
The organelles allow the creation of different chemical environments within one cell, and furthermore, by their structure they increase the area available for surface reactions which are all-important in biological systems. A description of the various organelles is given in the 14th edition of this book and Fig. 18.1C illustrates some aspects of their interdependence in the normal functioning of the cell. The molecular structures of these bodies have been extensively studied and details will be found in standard botanical texts.
Some basic metabolic pathways appear to be similar in both plants and animals, whereas others are more restricted in their occurrence. It is to the secondary plant products (i.e. those not necessarily involved in the essential metabolism of the cell) that the majority of vegetable drugs owe their therapeutic activity and so it is in these that pharmacognosists are particularly interested. However, as illustrated in Fig. 18.2, the production of these secondary metabolites is dependent on the fundamental metabolic cycles of the living tissue and so a brief indication of the latter will also be given; for fuller accounts of these, the student should consult a standard work on plant biochemistry.
ENZYMES
An enzyme usually acts on one substance or class of substances, since it is specific for a particular atomic group or linkage. Specificity, however, varies; lipases are, in general, not highly specific, whereas fumarase acts only upon L-malate and fumarate, while D-malate is a competitive inhibitor of fumarase. Enzymes are also stereo- and regio- specific in their actions and, as it becomes possible to prepare more rare examples, organic chemists are becoming increasingly aware of enzyme potential for carrying out single-step transformations with complete stereochemical exactitude, an aspect important in the synthesis of many drugs. An enzyme will convert many thousand times its own weight, and the gradual diminution in activity which takes place is probably due to secondary reactions which bring about destruction of the enzyme.
New horizons for the study of the enzymology of secondary metabolism have now opened up as a result of advances in gene technology. In suitable instances, by cloning, the cDNA responsible for an enzyme’s synthesis can be expressed in another organism such as a bacterium and large amounts of enzyme prepared. By conventional methods only very small amounts of purified enzyme could be obtained from the original plant material. Of particular interest has been the isolation, characterization and cloning of the enzyme strictosidine synthase. This governs the key reaction for the commencement of the biosynthesis of the very many monoterpenoid indole alkaloids, namely the condensation of tryptamine and secologanin to give 3alpha;(S)-strictosidine (see Chapter 26); for a review (126 references) on this enzyme see T. M. Kutchan, Phytochemistry, 1993, 32, 493.
Enzymes are colloidal in nature and consist of protein or contain protein as an essential part. They may be partly purified, and in some cases isolated, by the methods of protein chemistry (i.e. by fractional precipitation, dialysis and, more recently, gel and affinity chromatography, see Chapter 17). Most enzymes are soluble either in water or in dilute salt solutions and are precipitated by alcohol or acetone (acetone powders) and by high concentrations of salts. They are inactivated by heat, ultraviolet light and X-rays or by any treatment which brings about denaturation of proteins.
Chemical nature
their protein nature has been established. Their molecular weights are high, but vary from about 9000 (hydrogenase) to about 1 000 000. In many cases the component amino acids are known and in some cases (e.g. ribonuclease) the amino acid sequence within the molecule has been determined. During the isolation of an enzyme, the purified protein (apoenzyme) may be inactive but regains its activity in the presence of an essential coenzyme or activator, which may be organic or inorganic.
Coenzymes
Some enzymes which require the presence of smaller organic molecules, called coenzymes, before they can function are of very common occurrence and participate in a large number of important biochemical reactions. One group of coenzymes consists of esters of phosphoric acid and various nucleosides. The adenosine and uridine phosphates contain one basic unit each (mononucleotides); they serve to transport energy in the form of high-energy phosphate bonds and this energy is made available for biochemical reactions in the presence of the appropriate enzyme by hydrolysis of the bond. Thus, the terminal phosphate bond of the adenosine triphosphate (ATP) on hydrolysis to adenosine diphosphate (ADP) affords 50 000 J mol−1.
Riboflavine (Fig. 31.2) is a component of the two coenzymes flavin mononucleotide (FMN) and flavin adenine dinucleotide (FAD). They participate in the biological oxidation–reduction system, and FAD facilitates the transfer of H+ ions from NADH to the oxidized cytochrome system.
Other coenzymes are the decarboxylation coenzymes thiamine, biotin and pyridoxine (Fig. 31.2). Folic acid (Fig. 31.2) derivatives participate in enzymatic reactions which involve one-carbon fragment transfers.
Classification of enzymes
As more enzymes are isolated, it becomes important to have a precise scheme of classification and nomenclature. Many long-established names such as pepsin, prunase, diastase and names for enzyme mixtures, such as emulsin and zymase, continue in use. A step towards uniform nomenclature was made when they were denoted by the name of the substrate and the termination ‘-ase’. Classes of enzymes were named similarly. Thus, the general term ‘esterase’ includes lipases, which hydrolyse fats; chlorophyllase hydrolyses chlorophyll; etc. The 1961 Report of the Commission on Enzymes*
* This report has a numbered classification for each enzyme. For example, the enzyme present in garlic, alliine-lyase, is numbered 4.4.1.4. The first number denotes the fourth of the six main groups already mentioned and the other numbers further subdivisions. Thus:
made the recommendation that the chemical reactions catalysed be generally adopted for classification and nomenclature. This, of course, presupposes that the exact chemical reaction is known; see also the 1984 International Union of Biochemistry publication (London, UK: Academic Press) Enzyme Nomenclature and any later reports. Enzymes are classified into six main groups (Table 18.1).
Table 18.1 Classification of some enzymes.
Group | Trivial name | Systematic name |
---|---|---|
1. Oxidoreductases | Glucose dehydrogenase | β-D-Glucose: NAD(P)-oxidoreductase |
p-Diphenyl oxidase (lactase) | p-Diphenol: O2 oxidoreductase | |
Peroxidase | Donor: H2O2 oxidoreductase | |
Catalase | H2O2: H2O2 orthoreductase | |
2. Transferase | α-Glucan phosphorylase | α-1,4-Glucan: orthophosphate glucosyl-transferase |
3. Hydrolases | Lipase | Glycerol ester hydrolase |
Chlorophyllase | Chlorophyll chlorophyllidohydrolyase | |
Tannase | Tannin acyl-hydrolase | |
α-Amylase | α-1,4-Glucan 4-glucano-hydrolyase | |
Inulase | Inulin 1-fructanohydrolase | |
4. Lyases | Aldolyase | Ketose-l-phosphate aldehydelyase |
Decarboxylase | L-Tryptophan-decarboxylase | |
5. Isomerases | Maleate isomerase | Maleate cis-trans-isomerase |
6. Ligases (Synthetases) | Asparagine synthetase | L-Aspartate: ammonia ligase (ADP) |
Oxidoreductases
As any oxidation implies a simultaneous reduction, the names ‘oxidase’ and ‘reductase’ may be applied to a single type of enzyme. Eleven different groups of oxidoreductases are recognized. Many oxidases (that is, enzymes that utilize molecular oxygen as acceptor) convert phenolic substances to quinones. They act on guaiacum resin to produce a blue colour which is used as a test for their detection. Oxidases include laccase (1.10.3.2), present in lac, and ascorbate oxidase (1.10.3.3), which is widely distributed in plants. Oxalate oxidase (1.2.3.4), a flavoprotein present in mosses and the leaves of higher plants, oxidizes oxalic acid into carbon dioxide and hydrogen peroxide. Peroxidases (1.11) are distinguished by the fact that they use hydrogen peroxide and not oxygen as the hydrogen acceptor. Catalase (1.11) must be regarded as an exception, since it catalyses the decomposition of hydrogen peroxide. An oxidoreductase of the morphine biosynthetic pathway (Chapter 26) has recently been purified and characterized; it catalyses the stereoselective reduction of salutaridine to 7(S)-salutaridinol using NADPH as cosubstrate.
Hydrolases
These include many different types, of which the following are some of pharmaceutical importance.
Isomerases
Two enzymes important in pathways leading to medicinally important metabolites are isopentenyl diphosphate (IPP) isomerase (EC 5.3.3.2) amd chalcone isomerase (EC 5.5.1.6). The former is involved in the isomerization of IPP and dimethylallyl diphosphate (Fig. 18.18), an essential step in the formation of terpenoids, and the latter is a key enzyme catalysing the isomerization of chalcones to their corresponding flavanones (Fig. 18.11).
PHOTOSYNTHESIS
had been accepted for many years.
ATP is a coenzyme and the high energy of the terminal phosphate bond is available to the organism for the supply of the energy necessary for endergonic reactions. The Hill reaction produces free oxygen and hydrogen ions which bring about the conversion of the electron carrier, NADP, to its reduced form NADPH (see ‘Coenzymes’).
C3 plants
The elucidation of the carbon reduction cycle (Fig. 18.3), largely by Calvin and his colleagues, was in large measure determined by methods dependent on exposing living plants (Chlorella) to 14C-labelled carbon dioxide for precise periods of time, some very short and amounting to a fraction of a second. The radioactive compounds produced were then isolated and identified. In this way a sequence for the formation of compounds was obtained. 3-Phosphoglyceric acid, a C3 compound, was the compound first formed in a labelled condition but it was only later in the investigation, after a number of 4-, 5-, 6- and 7-carbon systems had been isolated, that ribulose-1,5-diphosphate was shown to be the molecule with which carbon dioxide first reacts to give two molecules of phosphoglyceric acid. An unstable intermediate in this reaction is 2-carboxy-3-ketopentinol.
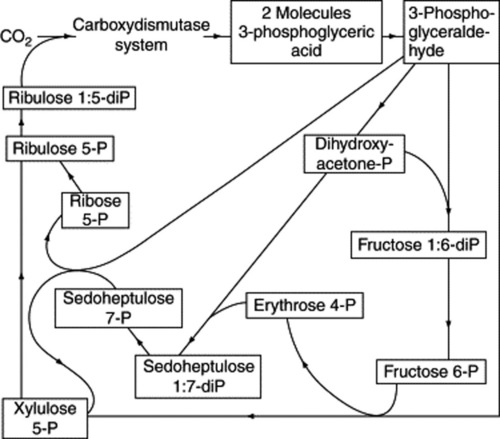
Fig. 18.3 The path of carbon in photosynthesis (after Calvin); the formulae of the sugars involved are given in Chapter 20.
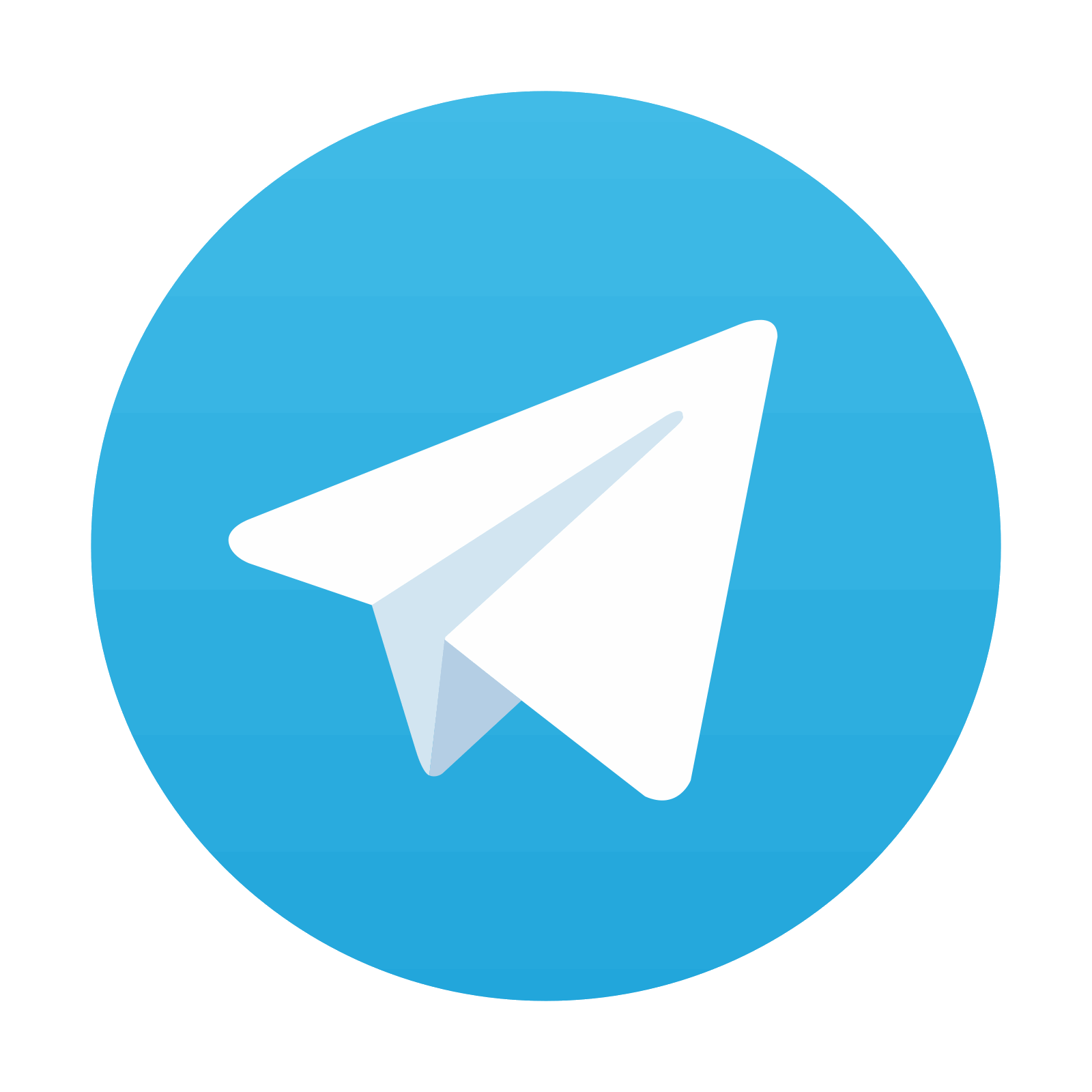
Stay updated, free articles. Join our Telegram channel
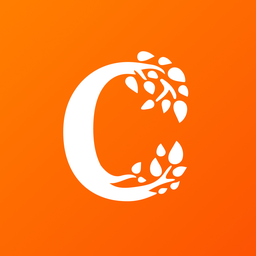
Full access? Get Clinical Tree
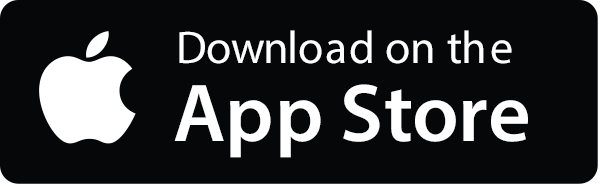
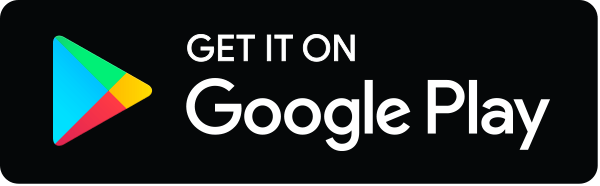