Chapter 1 LABORATORY MEDICINE
Introduction
Diagnostic disease-oriented laboratory testing. Such testing is usually ordered by the attending physician and is part of the initial work-up on almost all patients. These tests are routinely performed in all hospitals and ambulatory medical facilities.
Pretreatment assessment of the patient’s condition. Laboratory tests are routinely performed for all persons entering a hospital for medical, surgical, or emergency treatment.
Follow-up after treatment. Patients treated in hospitals or ambulatory clinics are regularly tested to determine the effects of treatment. For example, diabetics treated with insulin must regularly monitor their blood sugar levels to determine if their dosage of insulin or other medications is correct.
Screening for diseases. Screening tests are performed for populations at risk for a certain condition, such blood lipid profiles in patients with a history of familial hyperlipidemia, or after a person reaches a certain age, such as prostate specific antigen test in men older than 55 years.
Periodic monitoring of the state of health. Laboratory tests are typically part of the yearly medical examination recommended to monitor well-being. Additionally, they are almost invariably performed in special circumstances, such as pregnancy, infancy, and childhood. Many jobs require a pre-employment medical examination and school districts mandate preadmittance examinations that include routine laboratory testing. Together with physical examination, laboratory testing represents the most important part of the yearly medical examination performed by numerous health care providers.
Research. Laboratory tests are usually included in monitoring patients or normal persons being treated with new drugs (clinical trials) or those enrolled in research studies aimed at elucidating the pathophysiology of various diseases.
Laboratory Tests
Laboratory tests are performed on body fluids or tissue samples.
Laboratory tests can be classified as follows:
Routine tests performed in most hospital laboratories
Specialized tests performed only in specialized reference laboratories or under specific conditions and during complex medical procedures
Emergency tests performed on short notice and reported immediately to the ordering physician
Blood tests are performed usually in batteries, or panels, known as sequential multiple analysis (SMA-6, SMA-7, or SMA-12) (Table 1-1).
Table 1-1 Common Laboratory Panels
Blood may be submitted in tubes that either contain or do not contain anticoagulants. The tops of these tubes are color-coded to avoid confusion. Blood collected into a tube that does not contain an anticoagulant (red tubes) will clot, and the red blood cells, leukocytes, and platelets will separate from the serum (Fig. 1-1). The separation of blood cells from serum can be accelerated by collecting the blood in tubes that are not coated with anticoagulant. Such tubes contain a gel at the bottom that activates clotting and promotes the separation of the clot from the serum (“red/green,” also known as “tiger-top tubes” or “light green-mint tubes”). These tubes are used in the laboratories that perform automated testing. Serum contains all the normal minerals, enzymes, immunoglobulins, and proteins besides the coagulation factors, and is thus used for most routine laboratory tests.
Accuracy of a test Statistical term reflecting the capacity of the test to measure the true value of an analyte.
Acute-phase proteins Proteins that appear in increased concentration in blood in response to inflammation are called positive acute-phase proteins. This group includes C-reactive protein, transferrin, ceruloplasmin, fibrinogen, α1-antitrypsin, and several others. Proteins, like albumin, whose concentrations are is decreased in response to inflammation, are called negative acute-phase proteins.
Alkaline phosphatase Enzyme that hydrolyzes orthophosphoric esters, present in many cells of the body and in serum. Serum alkaline phosphatase is elevated in biliary obstruction, but it may also be derived from growing bones, bone undergoing remodeling (as in Paget’s disease), osteoblastic metastases, and bone-forming tumors.
Aminotransferase Also known as transaminase. A group of enzymes transferring amino groups from one amino acid to another. Alanine aminotransferase (ALT) and aspartate aminotransferase (AST) have clinical significance in liver function tests. ALT and AST are found in many other organs, and their serum concentration is elevated in other pathologic conditions as well (e.g., myocardial and lung infarctions).
Blood urea nitrogen (BUN) Blood nitrogen present in the form of urea. This test does not measure nitrogen included in proteins, accounting for approximately 18% of total blood nitrogen. BUN is elevated in renal failure. Since urea is a highly diffusible molecule its blood concentration falls rapidly after renal dialysis. BUN can be increased due to heart failure, shock, dehydration, and many other conditions and is a less specific marker of renal failure than elevated levels of creatinine.
Calcium A bivalent chemical element (Ca2+) present predominantly in the extracellular compartment in ionized form or in the form of salts. It is essential for many cellular functions. Hypercalcemia may be caused by hyperparathyroidism or several nonparathyroid-related conditions such a malignant tumors with metastases to bone. Hypocalcemia is less common and may be caused by reduced absorption or increased loss of calcium. Increased and decreased serum concentrations of calcium are associated with distinct pathophysiologic consequences.
Carbon dioxide (CO2) Gas produced in the body during oxidative respiration of cells and exhaled through the lungs into the atmosphere. Carbon dioxide along with bicarbonate (HCO3−) forms the most powerful of the body’s buffer systems in the blood. Hypercapnia (excessive CO2) and hypocapnia (decreased CO2) are mostly caused by respiratory and renal disturbances and are related to acid–base imbalance.
Chloride A monovalent chemical element (Cl−) mostly found in the extracellular fluid. In the serum it is a part of the anionic pool, but it can be also measured in urine and sweat.
Creatinine The end product of creatine degradation, which is excreted in urine. Serum creatinine is elevated in renal failure (azotemia).
Glucose Monosaccharide, formed from the degradation of glycogen, cellulose, and other complex carbohydrates. Blood glucose is elevated in diabetes mellitus and lowered in various conditions that cause hypoglycemia.
Lactate dehydrogenase (LDH) Ubiquitous enzyme involved in the removal of hydrogen from lactate. The concentration of LDH in blood is elevated in conditions marked by widespread cell destruction.
Lipoprotein A complex between proteins and lipids, found in the cell membranes and in the blood. By ultracentrifugation, blood lipoproteins can be separated into four categories: chylomicrons, very low density lipoproteins (VLDLs), low-density lipoproteins (LDLs), and high-density lipoproteins (HDLs). These lipoproteins carry other lipids, such as cholesterol, phospholipids, and triglycerides, throughout the body. Hyperlipidemia, which may be congenital or acquired, is associated with an increased risk for coronary atherosclerosis.
Phosphorus A monovalent chemical element (P), which is present predominantly in the extracellular space (in the form of monophosphates [PO4−] and diphosphates [PO42−]) or linked with calcium in complex salts that form the matrix of bones. Its metabolism is intimately linked with the metabolism of calcium. Inside the cells phosphorus is often attached to major macromolecules and energy-rich compounds such as adenosine triphosphate (ATP).
Plasma Liquid portion of the blood, containing water, minerals, proteins, lipoproteins, and monosaccharides. Serum is plasma minus the clotting factors.
Potassium A monovalent chemical element (K+) predominantly found inside the cells, but also in the extracellular compartment. For example the ratio of K+ inside the red blood cells and in plasma is 20:1. Hyperkalemia or hypokalemia are caused by several diseases and are associated with distinct pathophysiologic consequences, mostly pertaining to the contraction of cardiac and skeletal muscle.
Precision of a test Statistical term for the consistency with which the same result can be obtained when applying the same test repeatedly to the same sample.
Predictive value of a test Statistical term estimating the probability that a positive test result will identify a person with a disease (“true positives and false positives”).
Protein Group of macromolecules composed of amino acids. Proteins are essential for the maintenance of life, acting as structural elements, enzymes, membrane channels, and other vital cell components. They are also found in body fluids. Serum proteins can be divided by electrophoresis into two major groups: albumin and globulins. Hypoproteinemia may be caused by several diseases (most notably malnutrition, malabsorption of nutrients, and liver and kidney disease) and is associated with distinct pathophysiologic events.
Sensitivity of a test Statistical term for the capacity of a test to correctly identify individuals who have the disease (“true positives and false negatives”).
Specificity of a test Statistical term for the capacity of a test to correctly identify individuals who do not have the disease (“true negatives and false positives”).
The samples for specialized tests are often collected in a unique manner. For example diurnal variation of hormones can be measured on several specimens collected at predetermined times. The sweat test for cystic fibrosis requires stimulation of sweat production with pilocarpine. Enzyme deficiency, which is the hallmark of some inborn errors of metabolism, requires tissue samples containing cells. DNA analysis requires samples containing nucleated cells from a buccal smear or white blood cells.
Good laboratory tests must be precise (reliable) and accurate and have high sensitivity and specificity.
The accuracy of a test reflects how close the measured value is to the true value. An ideal test should have high precision and high accuracy. Like the target-shooting analogy shown in Figure 1-2, the outcome of each measurement should be as close as possible to the bull’s eye (“true value”) and the results should be as close together as possible (low scatter). These results can be graphically presented and typically have a bell-shaped (Gaussian) distribution with a low standard deviation from the mean. A test is unacceptable if it has high precision but does not reflect the true value (i.e., low accuracy). A test could have low precision but, if repeated several times, could have a statistically acceptable accuracy. Such tests have a wide standard deviation. Tests that have low precision and low accuracy are not used in clinical laboratories. Such tests may have a Gaussian distribution with a very high standard deviation or do not form a bell-shaped curve.
Good laboratory tests must have high sensitivity and specificity.
Specificity measures the incidence of “true negative” (TN) results; that is, normal values occur in all tested persons who do not have a disease—those who presented as “true negatives” or “false positives” (FP) (Fig. 1-3). Specificity is calculated according to the following formula:
Specificity (%) = [TN/(TN + FP)] × 100
Sensitivity (%) = [TP/(TP + FN)] × 100
The specificity and the sensitivity of a test can be adjusted by raising or lowering the cut-off point for a positive result (see Fig. 1-3). A test with high specificity will be always negative in health; that is, it will be negative for both FP and TN. Tests with a high sensitivity will be positive for all those who have a disease and will include all TP and FN.
Predictive value of a positive test (%) = [TP/(TP + FP)] × 100
Predictive value of a negative test (%) = [TN/(TN + FN)] ×100
Efficiency (%) = [(TP + TN)/(TP + TN + FP + FN)] × 100
Water and Sodium
Water, which accounts for approximately 60% of total body weight, is divided into two categories:
Intracellular volume (ICV), containing 70% of the total water
Extracellular volume (ECV) comprising the remaining 30% of total water, divided as interstitial compartment (22%) and plasma (8%)
Body water contains solutes that are unequally distributed in the ICV and ECV. Among these solutes are organic compounds, such as proteins, glucose, or lipids, and inorganics in the form of electrolytes and gases. Sodium (Na+) is the most important electrolyte in the ECV, and potassium (K+) is the most important electrolyte in the ICV (Fig. 1-4). Active maintenance of Na+ and K+ gradients across the cell membranes by energy-dependent pumps regulates the distribution of water in the body.
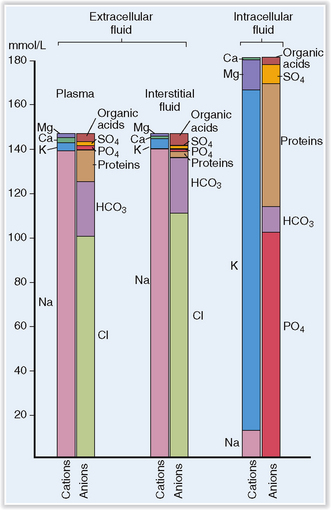
Figure 1-4 Concentration of electrolytes in plasma, interstitial fluid, and intracellular fluid.
(Redrawn from Edwards, CRW, Bouchier AD, Haslett C, Chivers ER [eds]: Davidson’s Principles and Practice of Medicine, 17th ed, Churchill Livingstone, Edinburgh, 1995, p 590.)
The concentration of sodium in the body depends on the amount of water and is controlled by kidneys and several hormones.
A regular diet contains more Na+ than the body needs, and the intestines can absorb unlimited amounts of this mineral. Thus intake of sodium is almost never a problem, and the body can adjust even if the exogenous salt is dramatically reduced. Most of the Na+ is excreted in urine. Small amounts of Na+ are lost in feces and sweat. Hence the excretion and loss equal the total intake (Fig. 1-5).
Sodium balance is under the control of several physiologic mechanisms:
Thirst. If one ingests too much sodium or loses too much water the osmoreceptors in the hypothalamus react to increase the osmolality of the plasma, thereby activating the thirst center. Intake of water brings down the osmolality of the plasma, ending the sensation of thirst.
Antidiuretic hormone (ADH, or arginine vasopressin). Increased osmolality caused by a loss of water stimulates the release of ADH from the hypothalamic center. ADH stimulates the resorption of water in the kidney until the osmolality of the plasma is reduced to normal. Depletion of the intravascular volume may stimulate thirst and ADH release independently of the changes in osmolality.
Aldosterone. Loss of ECV affects the glomerular filtration rate (GFR), causing a release of renin from the juxtaglomerular apparatus. Renin acts on angiotensinogen, which leads to an increased production of aldosterone from the zona glomerulosa of the adrenal cortex. Aldosterone promotes the exchange of Na+ for K+ or hydrogen (H+) in the distal renal tubules, causing retention of Na+ and expansion of the ECV.
Atrial natriuretic peptide (ANP). ANP stimulates the kidneys to increase the excretion of Na+ in urine.
Sodium plays a role in forming the electric charges across the cell membrane and in acid–base regulation and is the most important electrolyte regulating the osmolality of the extracellular fluid.
Acid–base balance. Sodium is an important cation that binds to several anions such as chloride, phosphates, and bicarbonates. Thus, it plays an important role in the maintenance of the acid–base balance.
Cell membrane polarity. Sodium is distributed differentially inside and outside the cell; it contributes to the formation of the electric charges across the cell membranes. The maintenance of the Na+ gradient across the cell membrane requires expenditure of energy, which is needed for the fueling of the Na+/K+ ATPase, the cell membrane pump, moving Na+ and K+ in or out of the cell.
Osmolality of body fluids. As the most abundant cation, Na+ is essential for the maintenance of the osmolality of body fluids in the normal range from 285 to 295 mOsm/kg. Thus, Na+ is essential for the maintenance of the volume of extracellular fluid (ECF), and indirectly also the volume of intracellular fluid. Even a small increase of osmolality of ECF stimulates osmoreceptors, which initiate thirst, ADH release, and water reabsorption in the kidneys. Reduced osmolality owing to overhydration reduces water intake and inhibits ADH secretion, allowing the excretion of water in urine.
Sodium concentration is the primary determinant of serum osmolality.
Serum osmolality is measured to determine the water content of ECF and exclude the possibility of overhydration or dehydration. In such cases the measured osmolality corresponds to the calculated osmolality, and the difference is usually less than 10 mOsm/kg. When an osmolal gap (>10 mOsm/kg) occurs, the discrepancy between the measured and calculated osmolality is usually caused by the presence of a low-molecular-weight substance (e.g., alcohol, ethylene glycol) in the blood. The study of the osmolal gap is especially useful in patients who are comatose or suspected of intoxication. The most common causes of increased serum osmolality are listed in Table 1-2.
Table 1-2 Causes of Altered Serum Osmolality
INCREASED OSMOLALITY | DECREASED OSMOLALITY |
BUN, blood urea nitrogen; SIADH, syndrome of inappropriate antidiuretic hormone secretion.
Based on data from Bakerman S, Strausbauch P: Bakerman’s ABC’s of Interpretative Laboratory Data, 3rd ed, Interpretative Laboratory Data, Myrtle Beach, SC, 1998.
Hyponatremia may be classified as dilutional or depletional.
Hyponatremia is a common condition defined as a serum concentration of Na+ below 136 mmol/L. Mild hyponatremia is found in 2 to 3% of all hospitalized patients. In most instances it is just a sign of illness, reflecting the inability of cells to maintain the normal gradient and flux of electrolytes across the cell membranes (“sick cell syndrome”). Severe hyponatremia is usually a consequence of water intoxication.
Pathogenetically, hyponatremia may be classified as dilutional or depletional (Fig. 1-6).
Dilutional hyponatremia occurs under the following conditions:
Increased water intake. This may occur due to obsessive water drinking (neurotic polydipsia), or in some lung cancer patients who have the paraneoplastic syndrome of inappropriate ADH secretion (SIADH).
Infusion of water. Most often it is a consequence of inappropriate intravenous infusion of fluids, but occasionally it my be also related to massive use of enemas.
Decreased excretion of water. This may be a consequence of heart failure or renal failure. In both conditions water is retained in the extracellular spaces.
Hypoproteinemia. Inadequate production of serum proteins in end-stage liver disease (cirrhosis) or a loss of proteins in nephrotic syndrome or chronic protein-losing gastroenteropathy results in hypoalbuminemia. Since albumin concentration is partly responsible for the oncotic pressure that keeps fluids inside the blood vessels, hypoalbuminemia leads to a shift of water from the vessels into the interstitial spaces and consequent hypovolemia. This loss of fluid triggers the ADH and the renin–angiotensin–aldosterone response, resulting in a net retention of water and dilutional hyponatremia.
Shift of water from cells into the ECV. This occurs in hyperglycemia of diabetes mellitus, paraproteinemia of multiple myeloma, or hyperlipidemia. In all these conditions, osmotically active substances in the blood and extracellular fluids cause a shift of fluids from the intracellular to the extracellular compartment. The total amount of Na+ remains the same but it is diluted by the influx of water into the ECV.
Syndrome of inappropriate antidiuretic hormone secretion (SIADH). ADH may be secreted by some tumors. Such a paraneoplastic syndrome may cause “water poisoning” due to excessive retention of water in the kidneys under the influence of ADH.


Symptoms of hyponatremia depend on the concentration of sodium and the way it has developed.
Death, if it occurs, is usually related to the underlying disease rather than hyponatremia itself.
Hyponatremia may be associated with increased total body water levels, relative increase in extracellular water, loss of Na+ in excess relative to body water or increase in total body water in excess to increased Na+ (Table 1-3).
When the plasma volume is reduced by 5% in chronic hyponatremia, ADH is released in spite of the osmoreceptor-generated inhibitory effects on its secretion. Due to the effects of ADH in chronic hyponatremia the volume of urine is reduced and it will be concentrated.
Hypernatremia is clinically almost always a consequence of dehydration.
Water loss can occur through the kidneys, GI tract, or the skin:
Renal loss of water. Hypernatremia is a symptom of diabetes insipidus, which may be central or nephrogenic in origin. Central diabetes insipidus may be primary (i.e., related to the injury of the hypothalamus or the posterior lobe of the pituitary) or secondary to drug treatment (e.g., lithium). Nephrogenic diabetes insipidus is a consequence of end-stage renal disease. In the postsurgical period, the patient may develop renal tubular necrosis and lose excessive amounts of water relative to Na+, especially during the polyuric phase of tubular necrosis. Other iatrogenic causes of hypernatremia include the use of loop and osmotic diuretics.
Gastrointestinal loss of water. Water may be lost during diarrhea or prolonged vomiting, but also because of prolonged suction through a nasogastric tube. Osmotic cathartic agents like lactulose may cause water loss.
Dermal loss of water. Dermal loss occurs in extreme heat that evokes profuse sweating, but it is also encountered after extensive burns.
Excessive sodium intake or retention. Sodium retention occurs under the following conditions:
Adrenal cortical lesions. Most commonly adrenal cortical abnormalities are responsible for hypercortisolism, causing Na+ retention. Such hypercortisolism may be due to benign or malignant adrenal cortical tumors.
Corticosteroids. Long-term use of corticosteroids in the treatment of rheumatoid arthritis, systemic autoimmune diseases, or nephrotic syndrome may also cause sodium retention.
Infusion of sodium-rich solutions. This may occur while infusing sodium bicarbonate (NaHCO3) during resuscitation, hypertonic saline feeding, administration of NaCl-rich emetics or enemas, or intrauterine injection of hypertonic saline.
Clinical findings depend on the nature of hypernatremia.
Clinically, hypernatremia must be always evaluated in the context of hydration of the body. If hypovolemia is present, urinary or intestinal water loss must be suspected. In euvolemic patients central or nephrogenic diabetes insipidus, dermal or respiratory loss of water, or inadequate intake of water (hypodipsia) must be considered. In hypervolemic patients an excess of mineralocorticoids, such as occurs in primary hyperaldosteronism, is a possibility (Fig. 1-7).
Chloride
Chloride is the major extracellular anion and it is tightly linked to intake, excretion and metabolism of sodium.
Hyperchloremic metabolic acidosis. Depletion of HCO3− in metabolic acidosis is usually accompanied by formation of organic anions, which will replace the lost HCO3−. If this does not occur, the gap is filled with Cl−. Hyperchloremia in this condition is not accompanied by hypernatremia.
Hypochloremic metabolic alkalosis. Metabolic alkalosis caused by a loss of Cl− in the GI tract is associated with an anion gap that is filled with HCO3−. The concentration of Na+ is in the normal range, and the condition can be treated with Cl− infusion.
Potassium
Potassium (K+) is the principal intracellular cation.
Potassium balance is maintained primarily by the kidneys.
The normal diet contains enough K+ and thus on average 100 mmol of K+ is absorbed daily, 90 mmol is excreted by the kidneys, and the remaining 10 mmol is lost in feces and sweat (Fig. 1-8).
The kidneys can regulate the excretion of K+, although approximately 30 mmol/day is excreted as obligatory renal loss. Potassium is filtered through the glomeruli but is almost completely absorbed in the proximal tubules (Fig. 1-9). The concentration of K+ in the blood and the glomerular filtration rate (GFR) regulate K+ excretion, because these two factors determine the amount of K+ available in the interstitial fluid of the kidneys for the exchange that occurs in the distal tubule and the collecting duct.
Potassium reenters from the interstitial fluid into the cells of the distal tubules and collecting duct. A small part of it is actively secreted into the lumen, but mostly K+ enters passively through diffusion. This diffusion occurs in response to the active reabsorption of Na+ from the lumen of tubular and ductal cells. Active Na+ reabsorption generates an electric membrane gradient, and K+ and hydrogen ions (H+) cross the membranes to neutralize these electric charges. Aldosterone promotes secretion of K+ in the distal tubule but also promotes, reabsorption of Na+, thus stimulating the sodium–potassium exchange (i.e., the excretion of K+ in urine). Remember that aldosterone secretion is stimulated by the renin–angiotensin system, but can also be increased directly by a high intake of K+.
Intercellular potassium concentration depends on the transmembrane exchange between the extracellular and intracellular pool of potassium.
The concentration of K+ inside the cells is an order of magnitude (approximately 25 times) higher than in the interstitial fluid. Such a huge gradient can be maintained only by the constant work of the sodium–potassium adenosine triphosphatase (Na+/K+ ATPase), which requires a huge expenditure of energy. Cell injury caused by ischemia or toxins may reduce the energy supply or impair the function of ATPase, resulting in a net increase of the efflux of K+ from the cells (Fig. 1-10).
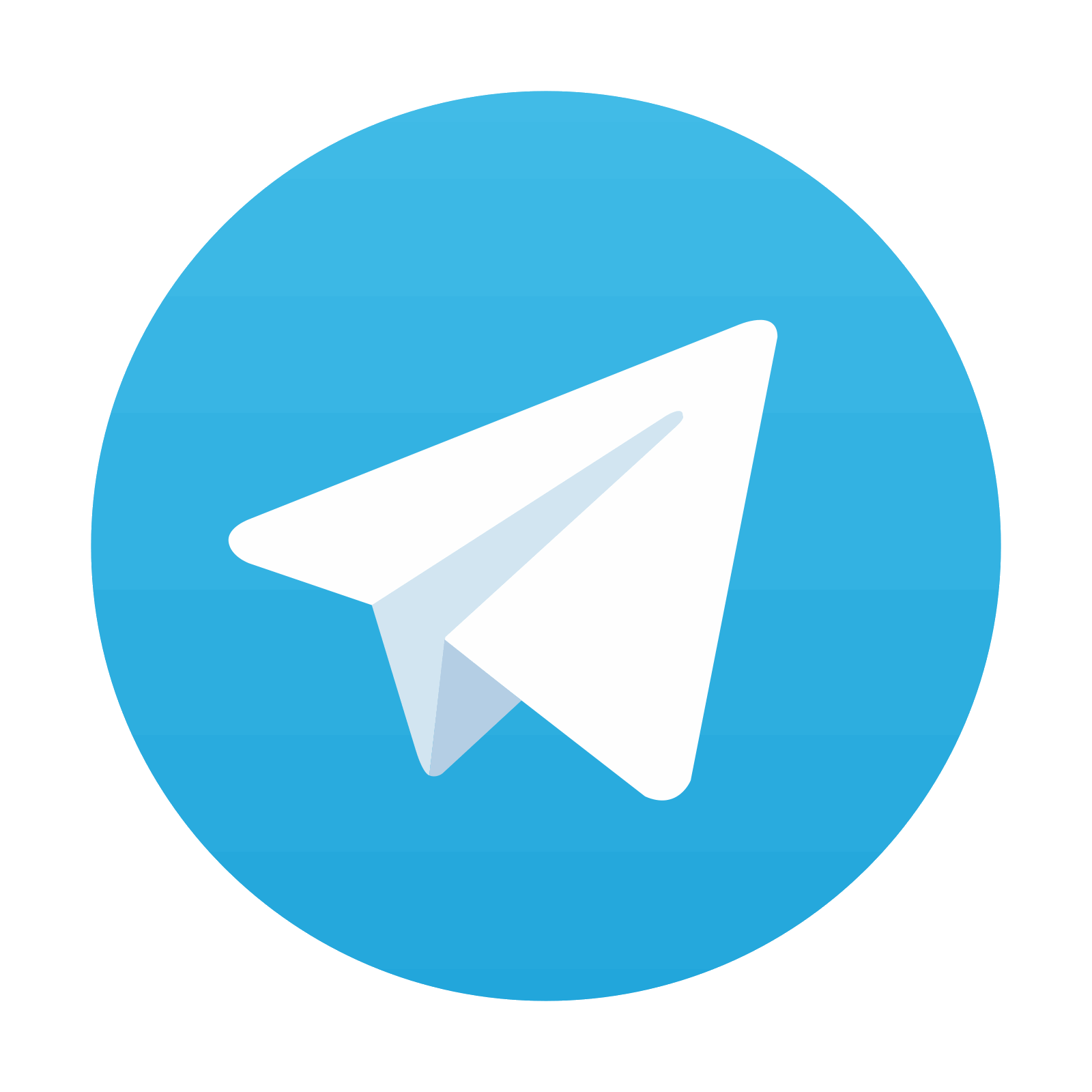
Stay updated, free articles. Join our Telegram channel
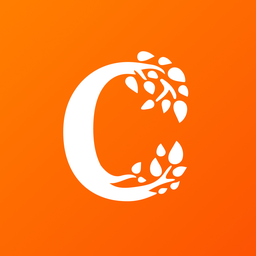
Full access? Get Clinical Tree
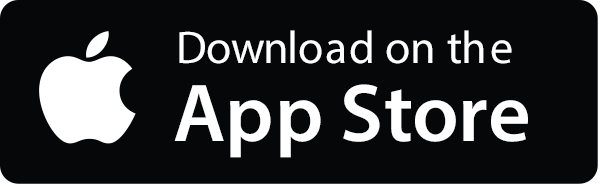
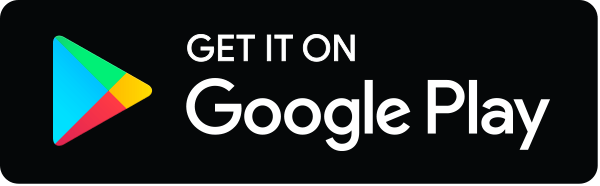