Fig. 10.1
Microparticle analysis procedure
The majority of the microparticles can be isolated through filtration using filter appropriate for the planned analysis. A gold-coated membrane filter (Rapid-ID, Berlin, Germany) facilitates FTIR-microscopic analysis of the particle directly on the filter without the need to pick up the particle and transfer it to a potassium bromide (KBr) disc. A polycarbonate membrane filter (Millipore Inc. Billerica) is better for SEM/EDS analysis, as it has no strong gold signal to interfere with other element analysis on the EDS spectrum. If the microparticle is larger than 100 μm, it may be retrieved directly with an appropriate probe (tungsten probe or plastic spatula) under a stereomicroscope in a laminar flow hood in a clean room (Class 100). The microparticles that are retained on the filters are then examined with a stereomicroscope to record their size and morphology, followed by FTIR-microscopic analysis. If they can be identified definitively by FTIR at this step (especially if they are organic in nature), further SEM/EDS analysis may not be required. However, for metallic and inorganic materials, SEM/EDS analysis is a must as these materials may not exhibit appropriately detailed FTIR or Raman spectra. Even for organic or polymeric materials, SEM/EDS analysis can provide additional information regarding the elemental composition of the materials to substantiate the vibrational spectroscopic analysis. In some cases the microparticles inside the glass container may be identified using in situ Raman microscopy (Cao et al. 2009, 2010). If the samples are amenable to in situ Raman analysis, this will be the ideal situation as Raman microscopy can provide identification without the need to open the container to isolate the microparticle and is a nondestructive technique.
For complex microparticles that are composed of multiple components, the above-mentioned techniques may not be sufficient to provide a definitive determination, and additional chromatographic and spectroscopic techniques such as inductively coupled plasma mass spectrometry (ICP-MS), gas chromatography (GC), high-performance liquid chromatography (HPLC/MS), and nuclear magnetic resonance (NMR) spectroscopy may be required. This may eventually lead to a full-scale comprehensive trace material analysis, which is beyond the scope of this chapter.
10.2.2 Optical Microscopy
Optical microscopy is the principal technique to observe and examine microparticles contained in a primary container. Optical microscopy is used to visualize the micron particles, in order to manipulate, pick up, and then transfer them for further FTIR and Raman analysis. Three major optical microscopes are commonly employed as the first step for microparticle analysis. A Carl Zeiss Stemi 2000C stereomicroscope can be employed to take pictures of microparticles that have been isolated onto filters. An Axioskop 2 MAT polarized microscope (Carl Zeiss Inc, Peabody) is employed to examine microparticles that may have birefringence such as liquid crystal polymer fiber. The polarized microscope can help to identify some materials that have a distinct birefringence such as cotton cellulose fibers, based on their optical properties. For in situ examination of microparticles contained inside the prefilled glass syringe and large-size glass vials, a long working distance and high-resolution optical microscope is required. These requirements are fulfilled with a Keyence high-magnification optical microscope VHX-600 or similar instrument (Keyence, Itasca), which can take high-magnification-quality images of very tiny particles in situ. The three microscopes are selectively employed according to the specific needs of investigations and the type of containers and samples being analyzed.
10.2.3 Vibrational Microspectroscopy
FTIR and Raman microscopy are two vibrational microspectroscopic techniques that provide complementary information on the molecular structure of materials (Humecki 1995; Li et al. 2009; Wen 2007; Wen et al. 2008). They are the result of a marriage between vibrational spectroscopy and optical microscopy. The combination of a microscope and a vibrational spectrometer offers the power to focus easily on a specific microparticle using the microscopic unit and then to record the vibrational spectrum of this selected particle with the spectrometer. They are the primary techniques for determining the identities of microparticles composed of polymeric or organic compounds at the molecular level. Some inorganic materials can also be examined by FTIR and Raman microscopy if they exhibit strong vibrational spectra.
The principles of FTIR and Raman spectroscopy are detailed in Chap. 3. FTIR-microscopy is generally preferred as the analytical tool for microparticle analysis for particles that have been isolated on a filter as the FTIR-microscope has been developed into a very sophisticated tool that is relatively easy to operate. Moreover, a large FTIR database of reference spectra with more than a quarter of a million compounds is now available from Bio-Rad Know-it-all (Shermann and Brodbelt 2002) that can be used to aid in particle identification. For microparticles composed of a single component, the FTIR spectrum of the microparticle is compared with the reference FTIR spectral library; if a perfect match is found to a reference spectrum from the library, the identity of the microparticle can be established. In cases where there is no perfect match, the chemical and structural information from a set of best matching reference spectra could still provide information on the category or structural family of the material, and to narrow down the possibilities and the direction the investigation should take. Further analysis relies on the experienced vibrational spectroscopist to interpret the FTIR spectra based on the structural chemistry using the empirical group frequency analysis approach, to determine the most likely composition based on the combination of functional groups and any knowledge relevant to the particles (Li et al. 2009; Smith 1999). In many cases, an identification of the type or category of a material can be very helpful in finding the root cause of the microparticles. This type of information can also be used to assess the potential chemical toxicity of the particles and for making decisions on product quality impact.
Raman microscopy is employed as a complementary technique to FTIR-microscopy. However, it has the advantage over FTIR-microscopy in that it can perform in situ analysis without the particle isolation procedure (Goldstein et al. 1992; Cao et al. 2009) for microparticles contained in glass vials and prefilled glass syringes. This is because the visible laser beam used in Raman can penetrate through the container without any interference from the glass or the aqueous solution. The glass (or plastic) containers strongly absorb infrared radiation and thus prevent FTIR measurements in situ. Another unique feature of Raman microscopy is that molecules that have rich electron density, such as conjugated aromatic compounds, show strong Raman bands (Chaps. 3, 13, and 16). Inorganic crystal material can exhibit strong Raman scattering in the low-frequency region as well due to the vibrational modes of the crystal lattice. The weakness of Raman microscopy is relatively low instrument sensitivity compared to FTIR-microscopy, resulting in longer acquisition time of a spectrum. Samples with a strong fluorescent background can also interfere with the ability to obtain a Raman spectrum. Currently the reference database for Raman spectra is also much smaller (15,000 Raman spectra) than the FTIR spectral database (a quarter of a million). Fortunately, the Raman database of organic and polymeric materials has been expanding rapidly in recent years with the continued improvement of the instrumentation, the increased ease of use, and the accumulation of Raman spectra of various materials in labs around the world. Raman microscopy will play an even greater role for microparticle analysis in the pharmaceutical industry in the near future.
10.2.4 Scanning Electron Microscope/Energy Dispersive Spectroscopy
SEM coupled with the energy dispersive X-ray spectroscopy (EDS) is a chemical microanalysis technique that utilizes X-rays emitted from the sample upon bombardment with an electron beam to characterize the elemental composition of the materials (Goldstein et al. 1992). The SEM function provides a high-resolution micrograph of the microparticle and the EDS provides a spectrum of the elementary composition. When the microparticle is irradiated by the electron beam, electrons are ejected from the atoms of the sample. The resulting electron vacancy is filled by electrons from higher orbit shells, and the characteristic X-rays of the element are emitted. The EDS spectrum consists of the characteristic X-rays of the elements, which is used for qualitative determinations of the elements in the sample (Goldstein et al. 1992). SEM/EDS is particularly useful for identification of microparticles from metallic and inorganic materials that do not produce molecular vibrational spectra. It is also a powerful tool for identifying composite materials of multiple elements. Another excellent feature of SEM/EDS instruments is the Cameo mapping function of an individual element in a complex microparticle composed of multiple elements. This feature can generate a distribution map of a selected element in the microparticle, showing whether the specific element is evenly distributed throughout the entire microparticle or concentrated in certain local areas. The combined use of optical microscopy, FTIR, and Raman microscopy as well as SEM/EDS offers a comprehensive toolbox that can be used for the analysis of the physical–chemical properties of the microparticles, in particular, for multicomponent materials.
10.3 Cases Studies
In the following sections, we will describe a few case studies of manufacturing NC investigation where visible microparticles were observed in primary containers of protein therapeutics.
10.3.1 Tungsten/Protein Particulates
Microparticles were initially observed in a protein drug product in prefilled glass syringes during a stability quality inspection, and the PFSs containing the particulates were then sent to the forensic lab for analysis. The top panel of Fig. 10.2 shows a picture of the microparticles retained on a membrane filter after isolation. The largest microparticle is about 100 μm and others are smaller. The bottom panel of Fig. 10.2 shows the FTIR spectrum of one representative particle, which is typical of protein FTIR spectrum. However, the FTIR spectrum exhibited a few extra distinctive bands at 954, 906, and 808 cm−1, respectively; the normal protein FTIR spectrum does not have sharp bands in this region. This suggested that there might be other components in the microparticle in addition to the protein. Subsequent analysis of the microparticle by SEM/EDS revealed that there was tungsten evenly distributed throughout the particle. Figure 10.3 displays the SEM micrograph and the EDS spectrum of a typical microparticle observed in the sample, showing the characteristic tungsten peaks at 1.775 and 8.396 keV, respectively. The identification of tungsten in the particle helped to explain the presence of the three small peaks at 954, 906, and 808 cm−1 in the FTIR spectrum; they can be assigned to tungsten oxide (Iacocca and Allgeier 2007). However, it was surprising to observe tungsten in protein particles in a PFS which is made of glass with no tungsten as its material components. The immediate question was, where did the tungsten come from and what was the role of tungsten in the formation of these protein particulates?
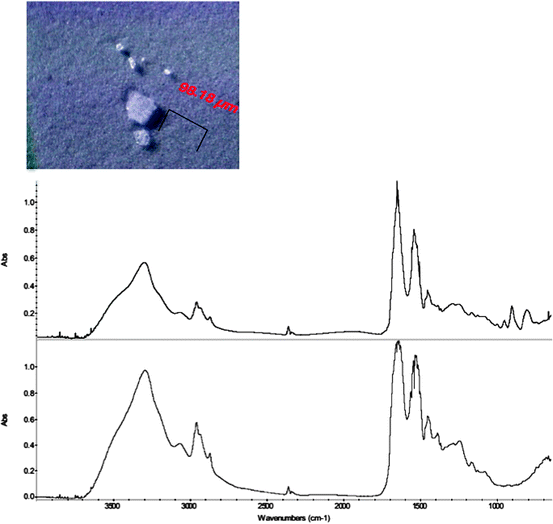
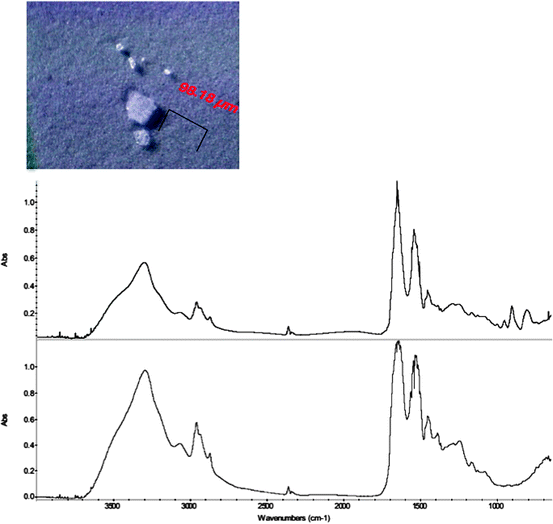
Fig. 10.2
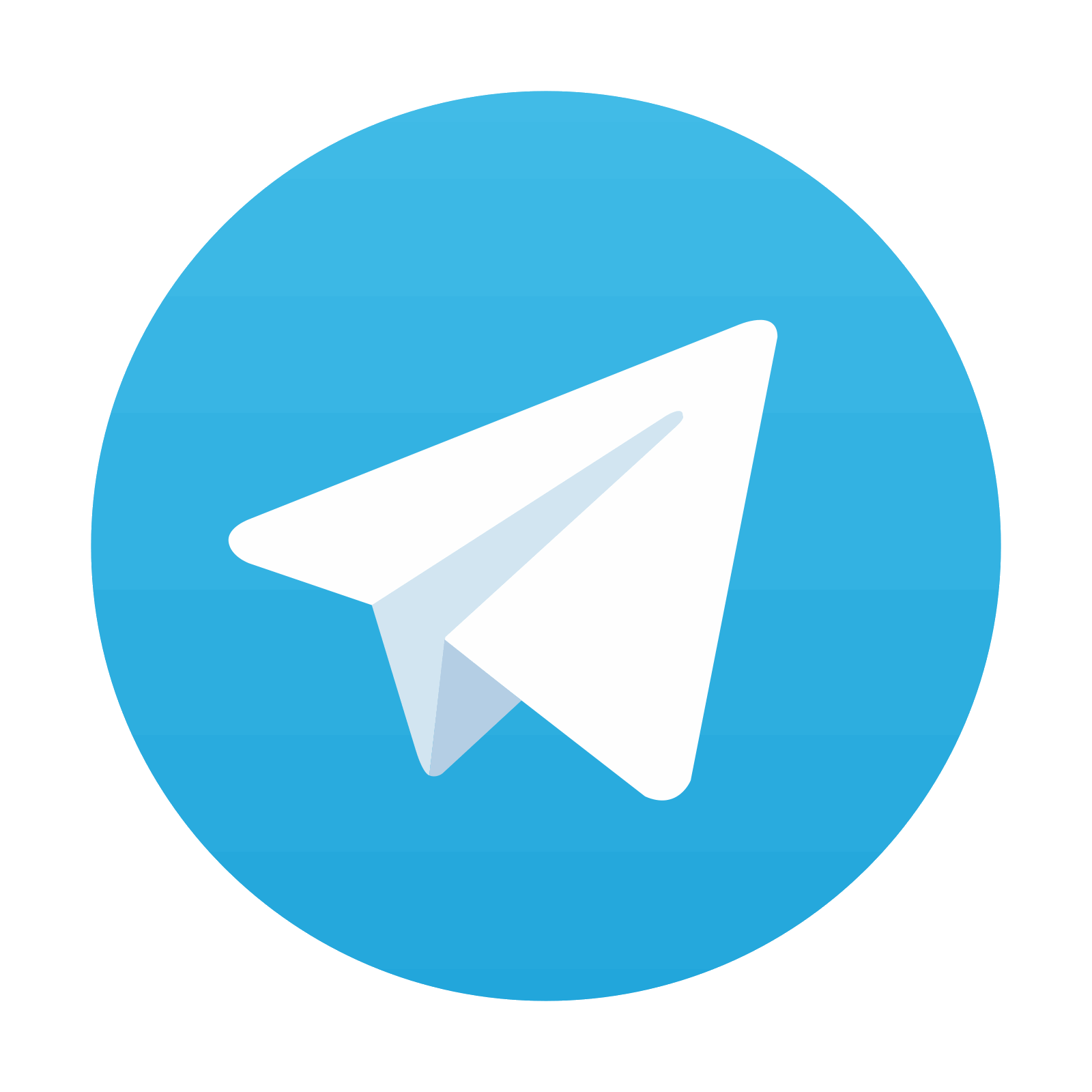
The micrograph of microparticles (top panel) and the FTIR spectrum (up trace) of a microparticle in a pre-filled syringe and the reference FTIR spectrum of a protein (lower trace)
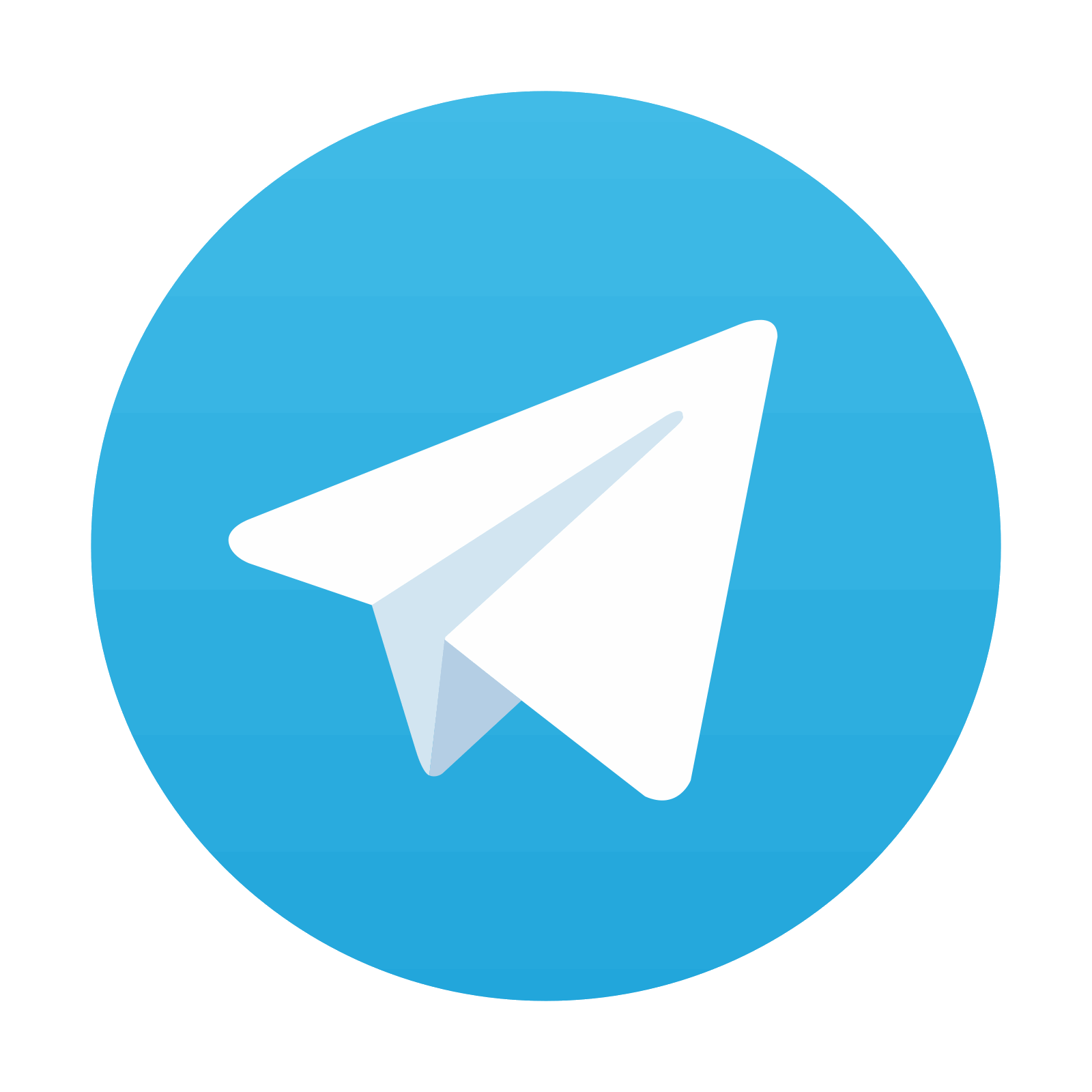
Stay updated, free articles. Join our Telegram channel
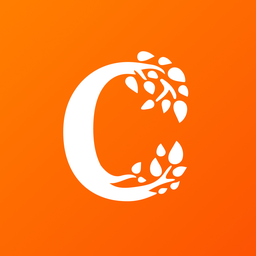
Full access? Get Clinical Tree
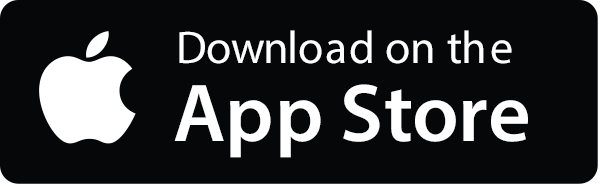
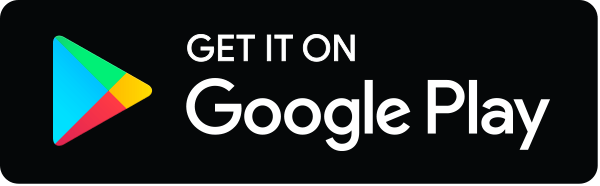
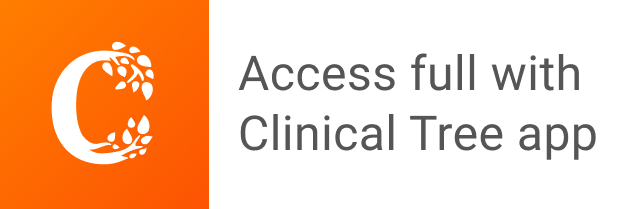