Fig. 6.1
Calcium signaling during fertilization and preimplantation development. Gametes and embryos are depicted at various stages of mammalian development to illustrate proposed roles for Ca2+ signaling. The process begins with gamete fusion and delivery of PLCζ by the sperm to initiate a series of Ca2+ transients responsible for the cortical reaction (exocytosis of the red vesicles shown), followed by extrusion of the second polar body and formation of two pronuclei. The approximate number of Ca2+ transients required for post-fertilization events are indicated to the right. Autocrine signaling and interactions with the reproductive tract during embryonic development extend the role of Ca2+ signaling. For example, PAF generates Ca2+ transients during the early cleavage stages that lead to CaMKII activation, which prevents apoptosis through downstream CREB activation. Speculation (?) suggests that the secretion of growth factors from the embryo and EGA could also be dependent on this signaling by Ca2+. Ca2+ signaling continues to regulate events leading to implantation. Examples are shown of proposed roles in cavitation and trafficking of integrins to the surface in response to LPA, CT, HBEGF and FN-induced integrin signaling as the blastocyst acquires competence for implantation. Details of these events are discussed in the article. Abbreviations: CaMKII, Ca2+/calmodulin kinase II; CT, calcitonin; CREB, cAMP responsive element binding protein; EGA, embryonic genome activation; FN, fibronectin; HBEGF, heparin-binding EGF-like growth factor; ITGA, integrin α subunit; LPA, lysophosphatidic acid; MAPK, mitogen activated protein kinase; PAF, platelet activating factor; PLC, phospholipase C
6.2 Intracellular Ca2+ Signaling During Fertilization
The role of intracellular Ca2+ signaling during fertilization, as illustrated at the top of Fig. 6.1, is well established and has been extensively reviewed elsewhere (Wakai and Fissore 2013; Ducibella and Fissore 2008). Fusion of sperm and egg triggers a series of cellular events known collectively as egg activation , which transitions the fertilized egg into embryogenesis. Activation of the metaphase II-arrested egg induces cortical granule exocytosis, molecular remodeling of the zona pellucida and plasma membrane, translational activation of stored maternal mRNA, resumption and completion of meiosis, extrusion of the second polar body, and pronuclear formation (Schultz and Kopf 1995).
Sperm-egg fusion, or experimental injection of a sperm, rapidly initiates PLC-dependent production of IP3 and activation of the ITPR to generate a large transient increase in the concentration of cytosolic free Ca2+, followed by a series of low frequency Ca2+ oscillations (Fig. 6.1) that last for several hours (Miyazaki et al. 1993; Cuthbertson et al. 1981). The production of these Ca2+ oscillations requires not only the initial burst of IP3, but also Ca2+ reuptake by the ER, Ca2+ influx across the plasma membrane, regulation of ER Ca2+ channels and Ca2+ buffering by other organelles (Wakai and Fissore 2013). The oocyte contains most isoforms of PLC, many which are capable of being activated by ligand-receptor interactions during fusion of the sperm and egg. However, IP3 production in the fertilized egg is initiated by PLCζ (Fig. 6.1), an isoform not expressed by the oocyte, but delivered by the entering spermatozoon (Swann and Lai 2013; Saunders et al. 2002). The decondensing sperm head releases PLCζ into the ooplasm where, unlike other PLC isoforms that utilize plasma membrane PIP2, it appears to hydrolyze PIP2 associated with small (< 1 μm), cytoplasmic vesicles. Accumulation of IP3 in the vicinity of cortical ER near the sperm head produces localized release of Ca2+ that propagates a rapidly growing wave of cytosolic free Ca2+ across the egg during the initial transient, but not during later oscillations (Deguchi et al. 2000). The necessity of Ca2+ signaling at fertilization is underscored by a subgroup of infertile men who produce morphologically normal sperm that fail to properly express PLCζ and are, thus, unable to activate eggs, even when injected (Yoon et al. 2008; Kashir et al. 2010).
One effect of Ca2+ signaling could be a switch in cellular metabolism in preparation for the egg to embryo transition. Several key mitochondrial enzymes are activated by Ca2+ to increase the production of ATP through oxidative phosphorylation (Campbell and Swann 2006), providing energy to initiate the embryonic developmental program and activate SERCA for restoring Ca2+ to the ER at the completion of each Ca2+ oscillation (Dumollard et al. 2004; Liu et al. 2001; Wakai et al. 2013). With each rise of cytosolic Ca2+, the release of ATP to activate the SERCA pump would terminate the transient and replenish the ER Ca2+ stores. The progression of egg activation involves an extensive network of protein kinase reactions, cytoskeletal rearrangements and de novo protein biosynthesis, which each require an infusion of energy.
Ca2+ signaling rapidly induces the cortical reaction and resumption of meiosis through mechanisms dependent on Ca2+-binding proteins. During oocyte maturation, cortical granules redistribute from the cytoplasm to the oocyte cortex adjacent to the plasma membrane where they are poised to secrete their contents once Ca2+ becomes available (Ducibella 1996). Thus, the Ca2+ transient initiated by PLCζ triggers the cortical reaction, which provides multiple blocks to polyspermy through the release of proteins that modify the zona pellucida and plasma membrane. The events of oocyte activation are driven to a large extent by protein kinases, which are directly or indirectly activated downstream of Ca2+ (Ducibella and Fissore 2008). For example, calmodulin (CaM) is a Ca2+-binding protein that can activate a number of protein kinases, including myosin light chain kinase, which is an immediate effector of cortical granule exocytosis during Ca2+ oscillations (Ducibella and Fissore 2008). Another downstream target of CaM, Ca2+/calmodulin kinase II (CaMKII), initiates cell cycle resumption in fertilized eggs by phosphorylating Emi2 to lift inhibition of the cyclosome complex, which contains E3 ubiquitin ligase that destroys cyclin B1, thus, relieving the inhibition of meiosis by maturation promoting factor or MPF (Nixon et al. 2002; Madgwick et al. 2005). Protein kinase C (PKC), activated by Ca2+ and the PLC product, DAG, appears to contribute to multiple processes that drive the resumption of meiosis in eggs (Ducibella and Fissore 2008). Additionally, PKC activation and translocation to the plasma membrane occurs with each Ca2+ rise to trigger SOCE, which amplifies the oscillation (Halet et al. 2004).
By recruiting Ca2+-activated signaling pathways to produce the physiological changes that drive meiosis, DNA synthesis, mitosis and other activities associated with egg activation , Ca2+ provides a ubiquitous signal that sequentially initiates highly diverse cellular events during the egg to embryo transition at fertilization. It has been demonstrated by experimentally inducing Ca2+ transients in unfertilized mouse eggs that different numbers of Ca2+ oscillations (Fig. 6.1) are required to activate different events during egg activation (Ducibella et al. 2002). It was reported that cortical granule exocytosis increased with each Ca2+ oscillation, beginning at the first one, while cell cycle resumption required 4 oscillations to begin, polar body extrusion required 8 and pronuclear formation 24. At the molecular level, the decrease in histone H1 kinase and MAPK activities required for cell cycle resumption were not observed until after 8 and 24 oscillations, respectively. Synthesis of new proteins from stored maternal message was first clearly observed in eggs receiving 8 Ca2+ oscillations and increased further with additional transients. There was also evidence of a return to the initial molecular state in eggs receiving less than the full number of Ca2+ transients. It has been suggested that Ca2+ signaling sequentially induces cellular processes through accumulating downstream reactions mediated by Ca2+-dependent protein kinases and other Ca2+-binding proteins (Ducibella and Fissore 2008).
The spatiotemporal complexity of Ca2+ signaling at fertilization reflects the diversity of cellular processes that are orchestrated throughout the course of egg activation , and the adverse outcomes associated with deviations from the normal pattern of oscillations (Ducibella and Fissore 2008). The programming of the fertilized egg to generate a series of transient increases in the intracellular Ca2+ concentration (Dumollard et al. 2002) provides an internal molecular clock that directs sequentially enfolding events of egg activation (Ducibella and Fissore 2008). Clearly, signaling downstream of Ca2+ is a high-level process that can operate through Ca2+-binding proteins and downstream pathways in other dynamic developing systems. The remaining sections will present evidence that intracellular Ca2+ does indeed continue to provide a master signal that regulates preimplantation embryogenesis spatiotemporally, and translates signals from the female reproductive tract to coordinate maternal and embryonic development.
6.3 Regulation of Cleavage Stage Development by Intracellular Ca2+
The Ca2+ oscillations induced at fertilization discontinue prior to first cleavage of the zygote (Cuthbertson et al. 1981), but the role of intracellular Ca2+ signaling does not end there. The success of preimplantation development depends on autocrine signaling by a number of trophic factors secreted from embryos . For this reason, embryos cultured as groups in small volumes promptly cleave, and produce blastocysts in greater number than embryos cultured singly in a large volume to dilute any accumulating secretions (Rappolee et al. 1988; Paria and Dey 1990; DeChiara et al. 1990; Palmieri et al. 1992; Schultz and Heyner 1992; Gardner et al. 1994; Wiley et al. 1995; O’Neill 1997). Embryos cultured in large volumes can, to some extent, be rescued by supplementing them with autocrine factors, including members of the epidermal growth factor (EGF) family and platelet-activating factor (PAF) , an ether phospholipid (1-o-alkyl-2-acetyl-sn-glyceryl-3-phosphocholine). Autocrine signaling is most critical at the 2-cell stage and is not mitogenic in nature, but rather ensures cell survival by reducing apoptosis (Brison and Schultz 1997, 1998; O’Neill 1998). While, there are most likely a large number of secreted autocrine factors required for proper early preimplantation development, intracellular Ca2+ constitutes a key common pathway for many growth factors and cytokines .
PAF signaling during preimplantation development has been examined extensively by O’Neill and co-workers during the past two decades (O’Neill et al. 2012) and provides an example of the central role of intracellular Ca2+ signaling during the period immediately after fertilization. Mouse zygotes or 2-cell embryos respond to PAF through a pertussis-sensitive GPCR (Honda et al. 2002) that can activate Gαq/11, Gαi, Gαo and Gβγ to regulate a wide variety of downstream signaling pathways, depending on their availability. In 2-cell embryos, PAF activates phosphatidylinositol-3 kinase (PI3K), most likely through Gβγ, to produce phosphatidylinositol-3,4,5-trisphosphate (PIP3). PIP3 is a docking site for pleckstrin homology domains that in early mouse embryos activates both PLC and a voltage-gated Ca2+ channel. Both intracellular Ca2+ release from the ER governed by the ITPR and Ca2+ influx across the plasma membrane though L-type channels are required to elevate the cytoplasmic free Ca2+ (Lu et al. 2003; Emerson et al. 2000). The Ca2+ transient persists for 5 min before the channels become desensitized. The channels recover after 90 min to 2 h, producing periodic Ca2+ transients that promote survival, according to studies in which the sources of Ca2+ were disrupted with inhibitors, or by using embryos from mice lacking the PAF receptor gene (Lu et al. 2004).
Ca2+ transients generated by PAF (middle of Fig. 6.1) activate CaM and dependent kinases, including CaMKII, which results in elevated phosphorylation of the cAMP responsive element binding protein (CREB) that can homodimerize or heterodimerize with another transcription factor, ATF1, to induce new transcription (Jin and O’Neill 2010). The phosphorylation of CREB is associated with its nuclear localization. There is not an absolute requirement of PAF for CREB phosphorylation, but rather an enhancement of signaling that contributes significantly to the development of competent embryos with adequate numbers of pluripotent stem cells at the blastocyst stage. Presumably, PAF signaling downstream of Ca2+, CaM and their target proteins could be complex and awaits further investigation. It has been suggested that PAF activation of CREB could contribute to the activation of transcription from the embryonic genome, possibly as one of many newly recruited or activated transcription factors working in combination with constitutively expressed factors to initiate embryonic gene expression during the 2-cell stage of murine development (O’Neill et al. 2012). Nothing is currently known about the role of Ca2+ signaling in embryonic genome activation, but it is interesting to speculate that activated transcription factors accumulate with repetitive cycles of intracellular Ca2+ transients to regulate this crucial event temporally, beginning at fertilization and continuing into embryogenesis.
Pathways downstream of intracellular Ca2+ that advance formation and development of the morula are less well understood. Extracellular Ca2+ regulates compaction through its interaction with adhesion molecules on the surfaces of blastomeres (Ducibella and Anderson 1975), setting the stage for cavitation. Regions of cell-cell contact that form adherens junctions are occupied by E-cadherin, a homophilic binding protein that requires Ca2+ ions, and is functionally disrupted by their removal (Hirano et al. 1987). While extracellular Ca2+ is required during compaction for surface adhesion molecules, including E-cadherin (Ohsugi et al. 1997), there is also evidence that intracellular Ca2+ is required to maintain compaction (Pey et al. 1998). Compaction is highly dependent on the activity of the Ca2+-requiring, cytoplasmic enzyme PKC (Winkel et al. 1990; Ohsugi et al. 1993, 1994). An intracellular Ca2+ transient is generated when morulae are decompacted by removal of extracellular Ca2+ or inhibition of PKC, CaM, voltage-gated Ca2+ channels or microfilaments (Pey et al. 1998). These treatments do not alter intracellular Ca2+ concentrations prior to compaction, indicating a specific association with the compacted state. Additionally, Ca2+ signaling does not cause these disruptions within the embryo, as induction of a Ca2+ transient with ionomycin has no detrimental effect on compacted embryos. One interpretation of these findings is that Ca2+ is released from binding proteins into the cytoplasm by disrupting cell shape and adhesion of the morula, suggesting that maintenance of the compacted state is dependent on regulatory Ca2+-protein interactions.
6.4 Regulation of Blastocyst Formation by Intracellular Ca2+
A role for Ca2+ signaling throughout preimplantation development was first hypothesized from studies of the teratogen ethanol (Armant and Saunders 1996). Ethanol causes fetal alcohol spectrum disorder subsequent to maternal alcohol consumption during the post-implantation period, leading to central nervous system defects, craniofacial deformities and fetal growth restriction (Hannigan and Armant 2000). High concentrations of ethanol can be toxic to embryos during preimplantation development, but after exposure of mouse embryos at the 1-cell or 2-cell stage to relatively mild concentrations of approximately 0.1 % (~ 25 mM) ethanol, in vitro development to blastocyst significantly increases towards the in vivo rate, with increased cell numbers and accelerated differentiation of the trophoblast cells to a migratory phenotype (Leach et al. 1993). Considering the known ability of ethanol to activate mouse eggs by elevating intracellular Ca2+ (Cuthbertson et al. 1981) and the role of Ca2+ signaling downstream of embryotrophic factors such as PAF (Emerson et al. 2000), it is feasible that ethanol operates through a similar Ca2+-mediated mechanism. Evidence supporting a role for Ca2+ signaling in advancing the program for blastocyst formation has now accumulated from studies conducted mainly in the mouse model.
Mouse embryos exposed to ethanol during culture from the 8-cell stage cavitate faster than control embryos (Stachecki et al. 1994b). Exposure for 5 min is as effective as a 24-h exposure or a brief exposure to Ca2+ ionophore, suggesting a rapid Ca2+ signaling mechanism. Indeed, real time monitoring with the fluorescent intracellular Ca2+ indicator fluo-3-AM demonstrates a Ca2+ transient immediately after exposure to 0.1 % ethanol that is independent of extracellular Ca2+ and persists for about 5 min. Chelation of intracellular Ca2+ by pretreating embryos with BAPTA-AM prevents elevation of intracellular Ca2+ and attenuates the acceleration of cavitation rates when 8-cell embryos are exposed either to ethanol or the Ca2+ ionophore, ionomycin (Stachecki and Armant 1996b).
The source of cytoplasmic Ca2+ in cavitating preimplantation mouse embryos has been examined. Ca2+ transients induced at the 8-cell stage by ethanol are attenuated in embryos pretreated with inhibitors of PLC, and PLC inhibition significantly delays fluid accumulation during blastocyst formation (Stachecki and Armant 1996a). The latter finding together with the observed delay of basal cavitation rates by BAPTA-AM treatment (Stachecki and Armant 1996b) reveals an explicit requirement for Ca2+ and IP3 signaling during this phase of embryonic development. Activation of the ITPR with thimerosal induces a Ca2+ transient in embryos, but the RYR agonist caffeine is without affect (Stachecki and Armant 1996b), consistent with a critical role for the ITPR. Treatment of semipermeabilized embryos with IP3, but not ryanodine, initiates a large Ca2+ transient that is blocked by BAPTA-AM pretreatment, demonstrating robust mobilization of Ca2+ stores through the IP3 pathway (Stachecki and Armant 1996b). Similar experiments have been conducted by microinjection of IP3 or ryanodine into mouse eggs with comparable results (Kline and Kline 1994), suggesting that this mechanism is retained from an earlier stage, although the extrinsic triggers responsible might differ. The concentration of intracellular Ca2+ and the rate of blastocoel formation were not increased by adding DAG, the other product of PLC enzymatic activity, nor did PKC inhibitors attenuate the acceleration of cavitation by ethanol, demonstrating that IP3 and Ca2+ are exclusively responsible for transmitting the observed stimulatory effects (Stachecki and Armant 1996a). Whereas PAF could provide the stimulus in zygotes and 2-cell embryos (Emerson et al. 2000), lysophosphatidic acid (LPA; Fig. 6.1, bottom left), another bioactive phospholipid produced in the uterus (Tokumura et al. 2000), generates PLC-dependent Ca2+ transients in 8-cell mouse embryos (Stachecki and Armant 1996a). Production of these and other embryotrophic factors by embryos and the reproductive tract could provide autocrine and paracrine stimuli, respectively, that activate PLC in vivo to advance the embryonic developmental program and coordinate it with that of the uterus .
Although PLC also generates DAG to activate PKC, cavitation of mouse morulae is not stimulated by supplementation with synthetic DAG or phorbol ester, and PKC inhibitors do not prevent ethanol-induced acceleration of cavitation (Stachecki and Armant 1996a). However, PKC inhibition does delay the basal rate of cavitation, indicating a role for PKC in homeostasis of the developing embryos. More critical in the acceleration of embryo cavitation is CaM (Stachecki and Armant 1996b). Inhibition of CaM by W-7 attenuates the acceleration of blastocoel formation after treatment with Ca2+ ionophore. Moreover, W-7 causes a dose-dependent delay in the basal cavitation rate, providing strong evidence for a requirement of this signaling pathway in normal development. CaM regulates a plethora of signaling and enzymatic activities through its interactions with other proteins (Lu and Means 1993), which in turn control cell proliferation, secretion and other critical functions for development. The role of CaMKII in advancing the cell cycle (Lorca et al. 1994) could regulate the timing of other developmental events that might be linked to the cell cycle. The generation of a Ca2+ transient clearly increases cell numbers at the same time as cavitation is stimulated (Stachecki and Armant 1996b). Protein secretion or trafficking events could be critical for advancing the developmental program and positioning the embryo for subsequent interactions with its microenvironment. The role of CaM in regulating these processes has long been appreciated (Chamberlain et al. 1995; Chen et al. 1999). The intricacies of Ca2+ signaling and its downstream networks (Ashby and Tepikin 2002) could be particularly effective for coordinating de novo gene expression with intracellular protein trafficking and secretory activities that regulate the developmental program. Additionally, CaM-independent activation of proteins by Ca2+ could also play a role in advancing preimplantation development . Clearly, there remains much to be learned about the regulation of blastocyst formation by Ca2+ downstream signaling.
6.5 Regulation of Trophoblast Adhesion by Intracellular Ca2+
As the blastocoel emerges and expands, the blastomeres complete differentiation into several new lineages. The mature blastocyst includes the pluripotent stem cells of the epiblast, a superficial portion of the inner cell mass that becomes the extraembryonic primitive endoderm, and trophoblast cells that form the first transporting epithelium, the trophectoderm (Wiley 1988), in the outermost layer of the embryo (Cockburn and Rossant 2010). With the increasing complexity of the embryo and the impending implantation of the blastocyst, cell-cell communication is of primary importance. A signaling system controlled by genes involved in cell lineage specification and the regulation of pluripotency directs mouse blastocyst development (Cockburn and Rossant 2010). While there have so far been no revelations regarding intracellular Ca2+ in the signaling pathways controlling differentiation of the various cell types that comprise the blastocyst, there is evidence that supports a role for Ca2+ signaling in interactions of mouse trophoblast cells with the maternal reproductive tract (Armant et al. 2000). With more sophisticated approaches for monitoring intracellular Ca2+ in real time, a role in cell lineage allocation of the preimplantation embryo could eventually come to light.
The molecular dialogue between trophoblast cells and the maternal environment (bottom of Fig. 6.1) includes paracrine signaling by factors secreted into the reproductive tract and juxtacrine signaling between endometrial tissues and embryonic cells that come into contact as implantation commences (Armant 2005). As a result of this communication, the uterine tissue becomes receptive to embryo implantation and the trophectoderm differentiates from a polarized epithelium into extravillous trophoblast cells capable of tissue invasion. Elevation of the intracellular Ca2+ concentration in mouse blastocysts with 0.1 % ethanol exposure rapidly alters gene expression, including the pluripotency regulator MYC and others responsible for rapid cell proliferation (Rout et al. 1997; Leach et al. 1999). This finding is consistent with observations that cell division rates increase in embryos after a Ca2+ transient (Stachecki and Armant 1996b; Leach et al. 1993). Expression of MYC is required for preimplantation development (Paria et al. 1992) and could be particularly important downstream of Ca2+ in the regulation of embryonic stem cells and trophoblast stem cells within the blastocyst. Intracellular Ca2+ signaling within the trophoblast cells is a key requirement in their phenotypic transformation and in coordinating the timing of this process with the ongoing development of the endometrium.
Secretions of the zona-enclosed blastocyst, as well as those of the endometrium, stimulate preimplantation embryonic development during transit from the oviduct into the uterus (Armant et al. 2000). The secreted products include agonists of both receptor tyrosine kinases and GPCRs, which activate a wide variety of downstream signaling pathways and second messengers, including Ca2+. Several experimental paradigms are available for examining the effects of embryotrophic factors on trophoblast differentiation using mouse blastocysts cultured in vitro (Armant 2006). These approaches have been used to demonstrate a critical role for Ca2+ signaling at the blastocyst stage, beginning with the observation that pharmacological induction of a Ca2+ transient not only accelerates development in vitro, but results in significantly improved implantation rates when treated blastocysts are transferred to pseudopregnant dams (Stachecki et al. 1994a).
Trophoblast differentiation is regulated by cross talk between GPCRs and receptor tyrosine kinases. Exposure of morulae or blastocysts to LPA significantly accelerates extravillous differentiation and this effect is attenuated by BAPTA-AM (Liu and Armant 2004). However, inhibition of ERBB receptor tyrosine kinases will prevent LPA stimulation of trophoblast development, suggesting that receptor cross talk is required in addition to the IP3-mediated release of Ca2+ from intracellular stores. The LPA receptor and other GPCRs are known to activate ERBB family receptors by a variety of mechanisms, including transactivation of ERBB1 and ERBB4 by the heparin-binding EGF-like growth factor (HBEGF; Fig. 6.1) (Umata et al. 2001). Indeed, antagonizing HBEGF inhibits LPA-mediated stimulation of trophoblast differentiation (Liu and Armant 2004). Moreover, intracellular HBEGF, monitored by immunofluorescence microscopy, is observed trafficking to the apical surface of the trophectoderm within 45 min after exposure to LPA. It is released from the surface within another 15 min. HBEGF is a type 1 transmembrane protein that requires cleavage by metalloproteinases for secretion of its extracellular domain, which is then free to bind ERBB1/4 during autocrine signaling (Holbro and Hynes 2004).
Calcitonin is a hormone of the parafollicular cells of the thyroid gland that is also produced in the uterus just prior to implantation in response to surging progesterone (Ding et al. 1994). Antisense inhibition of calcitonin mRNA expression in the uterus disrupts receptivity for blastocyst implantation (Zhu et al. 1998a, b). Like LPA, calcitonin receptors are coupled to G proteins that mobilize cytoplasmic free Ca2+ (Martin et al. 1995). In rodents, uterine expression of calcitonin begins on gestation day 3, increasing by day 4 (Ding et al. 1994), while its receptor is highly expressed by preimplantation embryos (Wang et al. 1998). Embryos first respond to calcitonin at the 4-cell stage by producing an intracellular Ca2+ transient. Blastocysts treated for only 30 min with calcitonin go on to form trophoblast outgrowths, a sign of trophoblast differentiation, significantly earlier than control embryos (Fig. 6.1). Additionally, calcitonin-treated blastocysts exhibit a precocious onset of fibronectin binding activity on the surface of the trophectoderm, and trafficking of the integrin subunit α5 (ITGA5) to the apical surface, both indicators of adhesion competence. Pretreatment of embryos with the intracellular Ca2+ chelator BAPTA-AM prevents both production of a Ca2+ transient and acceleration of trophoblast differentiation after calcitonin exposure (Wang et al. 1998).
The mechanism of HBEGF-induced stimulation of trophoblast development (Fig. 6.1) suggests a hypothesis to explain the requirement for its transactivation downstream of LPA. HBEGF accelerates trophoblast differentiation and induces ITGA5 trafficking in the absence of a GPCR agonist (Wang et al. 2000). Activation of the trophoblast is associated with, and dependent on, the production of a prolonged Ca2+ transient. Since the gamma isoforms of PLC (PLCγ1 and PLCγ2) can be activated by tyrosine phosphorylation (Meisenhelder et al. 1989; Margolis et al. 1989), it is possible that IP3-mediated release of Ca2+ from the ER produces the observed Ca2+ transient and acceleration of trophoblast differentiation; however, neither process is affected by HBEGF in the presence of a PLC inhibitor. Conversely, chelation of extracellular Ca2+ effectively blocks both the elevation of intracellular Ca2+ and the accelerated development (Wang et al. 2000). Furthermore, treatments with a panel of Ca2+ channel blockers demonstrated a requirement for N-type voltage-gated Ca2+ channels. It appears that HBEGF induces Ca2+ influx to sustain Ca2+ signaling and provide Ca2+ for replenishment of the ER at the end of the transient. The Ca2+ influx mechanism could therefore be essential after release of Ca2+ from the ER by GPCRs and might explain the cross talk between LPA or other GPCR agonists and the EGF signaling system. Voltage-gated Ca2+ channels have an important role during blastocyst development, demonstrated by implantation failure of blastocysts treated with high concentrations of endocannabinoids that inhibit voltage-gated channels (Wang et al. 2003).
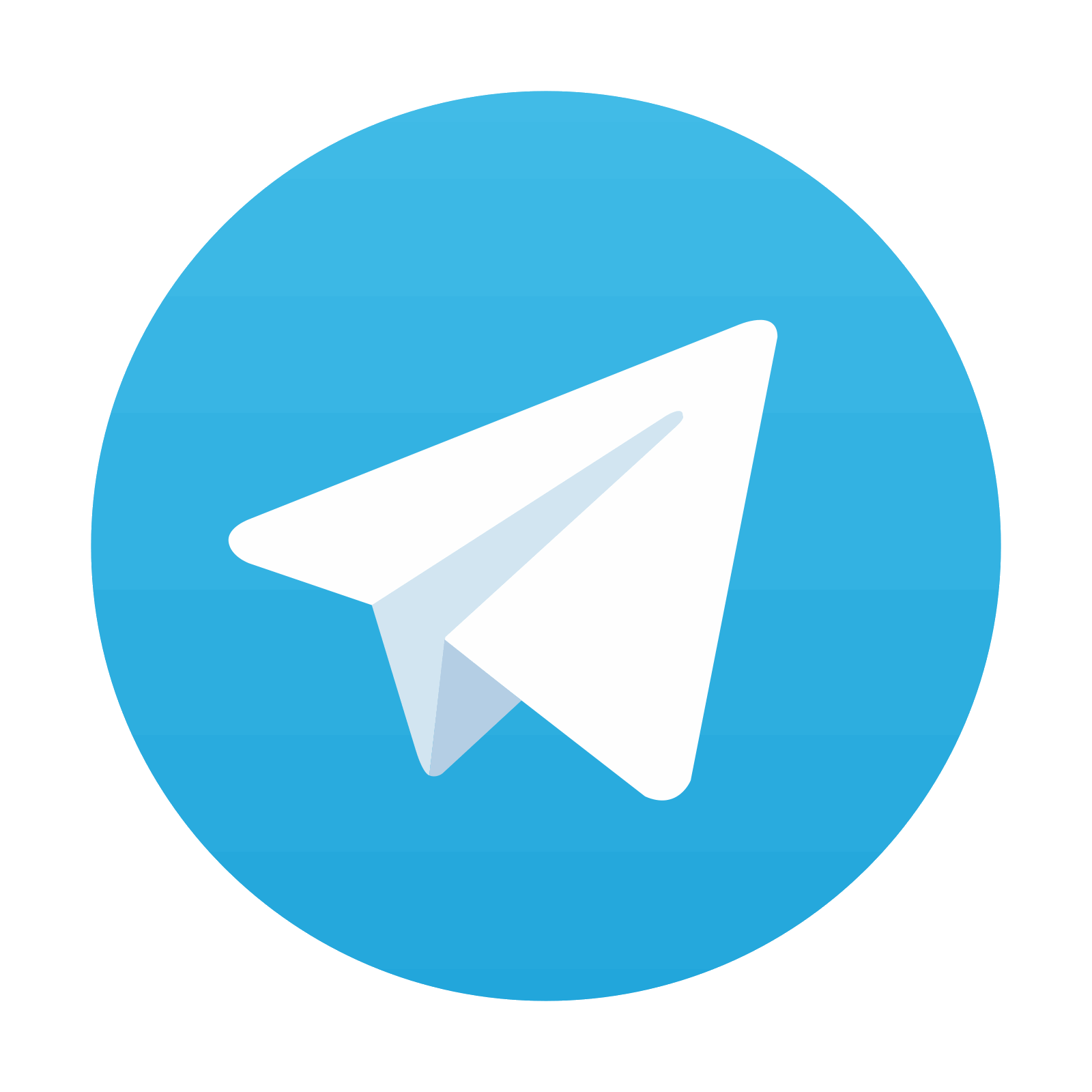
Stay updated, free articles. Join our Telegram channel
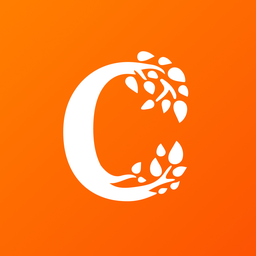
Full access? Get Clinical Tree
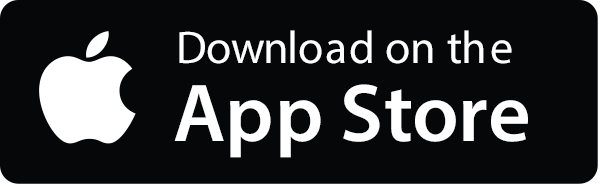
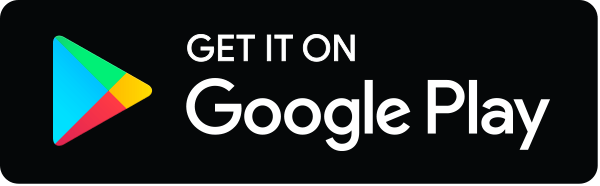