Chapter 16 Inherited metabolic diseases
Introduction
Many inherited diseases are known to be due to the genetically determined absence or modification of specific proteins. For example, in sickle cell anaemia, the protein is haemoglobin; in agammaglobulinaemia, antibody production is defective. However, in the majority of such diseases, the protein in question is an enzyme, and the effect is to cause a metabolic disorder. Other inherited metabolic diseases may be due to defective receptor synthesis (e.g. familial hypercholesterolaemia, which affects the receptor for low density lipoprotein) or to defects involving carrier proteins (e.g. cystinuria, in which renal tubular reabsorption of cystine is impaired). Whatever the cause, the clinical features of inherited metabolic diseases stem directly from the metabolic abnormalities to which they give rise. Although individually these conditions are rare (Fig. 16.1), they are of considerable significance; the consequences of many of them are potentially severe, but may, in some cases, be ameliorated if an early diagnosis is made and the appropriate treatment instituted.
Because they are individually rare, it is important for the clinician to have a high index of suspicion and actively consider the possibility that an illness may be caused by an inherited metabolic disease. Common clinical presentations of inherited metabolic diseases are indicated in Figure 16.2. Simple screening tests that should be performed when one of these conditions is suspected are discussed in Chapter 21. Most inherited metabolic diseases present in infancy and childhood (sometimes in association with specific events, e.g. weaning, puberty); their diagnosis and management is the province of paediatricians, albeit usually in close collaboration with the laboratory staff. With improving treatment, affected children with some conditions that hitherto were usually fatal in childhood are surviving into adulthood and being managed in dedicated adult metabolic clinics. Some inherited metabolic diseases usually present clinically only in adults, an important example being familial hypercholesterolaemia (see p. 248), although homozygotes for this dominantly inherited condition tend to present in their late teenage years and early twenties.
In a book of this size, it is only possible to discuss a selection of the many hundreds of inherited metabolic diseases that have been described. The ones that have been chosen are either among the more common, or illustrate important general principles with regard to presentation, diagnosis and management, or both. Many others are discussed in other chapters of this book (Fig. 16.3).
Effects of enzyme defects
Figure 16.4A shows a hypothetical metabolic pathway involving the synthesis of product D from substrate A by successive, enzyme-catalysed reactions through intermediates B and C. If the formation of B from A, catalysed by enzyme a, is rate limiting, as the first step unique to a metabolic pathway frequently is, then the concentrations of intermediates B and C will normally be low. The formation of product E from C, catalysed by enzyme c′, is normally a minor pathway, only a small amount of E being formed.
Decreased formation of the product
Decreased formation of the product of a reaction is the most obvious consequence of a lack of enzyme c (see Fig. 16.4B). If enzyme c is defective, D cannot be synthesized or may only be synthesized in small amounts. Clinical features will arise if product D has an essential function and there is no alternative pathway for its synthesis.
Accumulation of the substrate
Accumulation of the substrate (C) of the missing enzyme would also be expected (see Fig. 16.4C). If this is biologically active, clinical manifestations will result. Other, earlier substrates may also accumulate if the reactions prior to the one blocked are reversible. This will occur particularly if there is negative feedback by the product on an early reaction in the pathway because, with decreased formation of the product, feedback will be lost, thus reversing the inhibition and stimulating the formation of the intermediate substrates.
Increased formation of other metabolites
Increased formation of E, the product of a minor pathway, may occur if the concentration of C is increased as a result of the enzyme deficiency, the reaction being promoted by a mass action effect (see Fig. 16.4D). If product E is biologically active, a clinical syndrome will result.
Inherited Metabolic Disorders
Glucose 6-phosphatase deficiency
Glucose 6-phosphatase deficiency (glycogen storage disease type IA) exemplifies the development of a clinical syndrome due to lack of formation of the product of an enzyme-catalysed reaction. Glucose synthesis from glycogen or by gluconeogenesis is blocked (Fig. 16.5). Children with this disorder are prone to severe fasting hypoglycaemia, because their only source of glucose is dietary carbohydrate and the small amounts of glucose that can be liberated from glycogen by debranching enzyme.
Galactosaemia
Three enzyme defects can cause galactosaemia, and exemplify the production of a clinical syndrome due to the accumulation of a substrate of the missing enzyme. The enzyme galactose 1-phosphate uridyl transferase is required for the conversion of galactose to glucose 1-phosphate (Fig. 16.6), thereby allowing galactose to be incorporated into glycogen, converted into glucose or to undergo glycolysis. Absence of the enzyme in classic galactosaemia results in the accumulation of galactose 1-phosphate. The clinical features of the condition are thought to be due directly to the toxicity of this metabolite. In addition, the plasma concentration of galactose is increased and galactose is excreted in the urine. Infants with galactosaemia present with failure to thrive, vomiting, hepatomegaly and jaundice. Septicaemia, particularly due to Escherichia coli, is also common. Cataracts may be present as a result of the conversion of excess galactose to galactitol in the lens. There may also be hypoglycaemia and impairment of renal tubular function. Galactose is a reducing sugar, and a positive test for urinary reducing substances in an infant presenting with such symptoms raises the possibility of galactosaemia. Galactose (and lactose, present in milk) should be withdrawn from the diet pending a definitive diagnosis, based on measurements of galactose 1-phosphate uridyl transferase in erythrocytes. The response to treatment (continued exclusion of galactose from the diet) is monitored by measuring galactose 1-phosphate in erythrocytes. A case of classic galactosaemia is presented in Case history 21.5. Deficiency of the enzyme UDP-galactose 4-epimerase causes a similar clinical syndrome, but is much less common. Deficiency of the enzyme galactokinase prevents the phosphorylation of galactose and leads to an increase in the plasma concentration of galactose and thus to galactosuria. Because galactose 1-phosphate formation is blocked, this metabolite does not accumulate and, although cataracts may occur, the other clinical features of classic galactosaemia are not seen in galactokinase deficiency.
Phenylketonuria
Phenylketonuria (PKU) is another condition in which the accumulation of the substrate of the missing enzyme gives rise to a clinical syndrome. The enzyme concerned is phenylalanine hydroxylase, which hydroxylates phenylalanine to form tyrosine (Fig. 16.7).
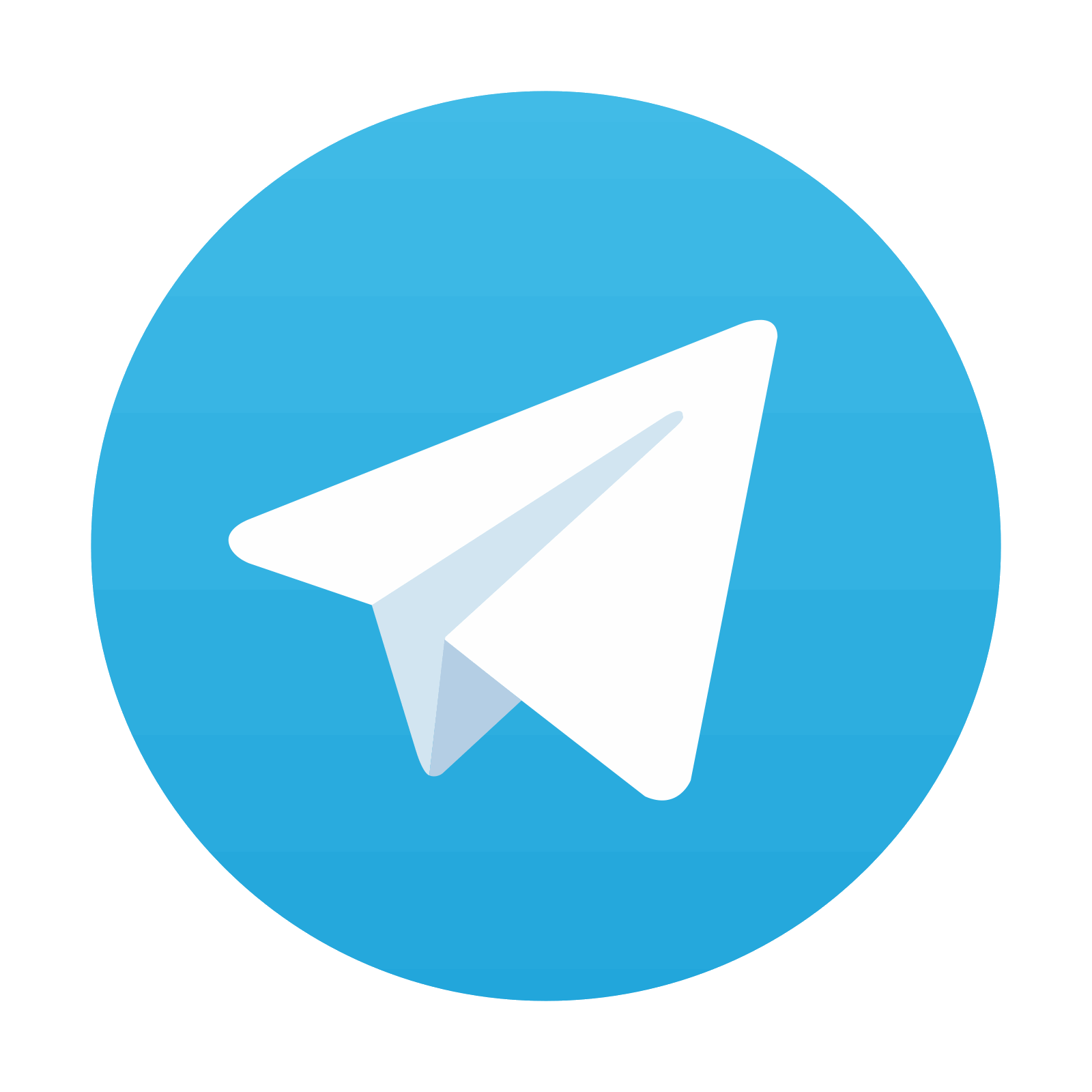
Stay updated, free articles. Join our Telegram channel
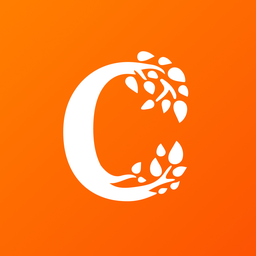
Full access? Get Clinical Tree
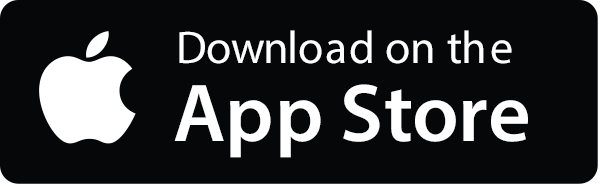
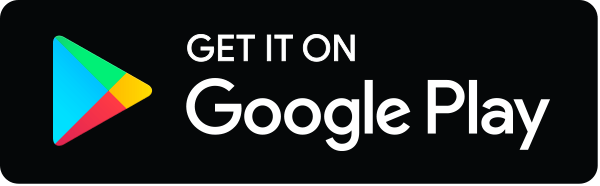