OVERVIEW
The daunting role of the immune system is to afford protection. It serves as a host defense system against infectious diseases and foreign (nonself) antigens. To accomplish this goal, the immune system is equipped with a rapid response mechanism, exquisite specificity, adaptability, an intricate regulatory network, and memory.
Over the past several decades, dramatic progress has taken place in the field of immunology. As a consequence, significant advances have been realized not only in the research realm but also in the diagnostic and clinical arena. These advances have allowed us to better understand how the immune system works and have provided insight into a variety of immune disorders, such as infectious diseases, allergy, autoimmunity, immunodeficiency, cancer, and transplantation. This information has led to better diagnosis, new treatment strategies, and improved management for patients with these disorders.
This chapter focuses on the basic principles of immunology, particularly as they relate to response to infection. More detailed discussions on the various aspects of the immune system are available in the reference section.
As the immune system defends the host against pathogens, it uses different recognition systems to effectively eliminate the invading pathogen or its products. A response generated against a potential pathogen is called an immune response. The first line of defense, which is nonspecific to the invading pathogen, is rapidly mobilized at the initial site of infection but lacks immunologic memory and is called innate immunity. The second defense system is called adaptive immunity. It is specific for the pathogen and confers protective immunity to reinfection with that pathogen. Adaptive immunity can specifically recognize and destroy the pathogen because lymphocytes carry specialized cellular receptors and produce specific antibodies. A protein that is produced in response to a particular pathogen is called the antibody, and the substance that induces the production of antibodies is called the antigen. In summary, the innate immune response is effective and critical in eliminating most pathogens. However, if this initial mechanism fails, the adaptive immune response is induced that specifically confronts the pathogen and establishes immunity to that invading pathogen. Hence, both systems interact and collaborate to achieve the final goal of destroying the pathogen.
INNATE IMMUNITY
Innate immunity is an immediate response to a pathogen that does not confer long-lasting protective immunity. It is a nonspecific defense system and includes barriers to infectious agents, such as the skin (epithelium) and mucous membranes. It also includes many immune components important in the adaptive immune response, including phagocytic cells, natural killer (NK) cells, Toll-like receptors (TLRs), cytokines, and complement.
Few microorganisms can penetrate body surfaces. These surfaces have an epithelial cell layer as their barrier, which is present in the skin, airways, gastrointestinal (GI) tract, and genitourinary tract. The epithelial cell layer has tight junctions and produces a number of powerful antimicrobial peptides that help provide protection against invading pathogens. Lysozyme is an example of an antimicrobial peptide that dissolves some bacterial cell walls. Another major peptide of innate host defense with antimicrobial properties is defensin. Defensins are positively charged peptides located primarily in the GI and lower respiratory tracts that create holes in bacterial cell walls and hence disrupt the bacterial membrane. Neutrophils in the small intestine contain azurophilic granules that house the α-defensins that are released following TLR activation, whereas epithelial cells in the respiratory tract secrete a different defensin, called β-defensin. The α-defensins have also been shown to possess antiviral activity. For example, α-defensins can inhibit HIV (human immunodeficiency virus) binding to the CXCR4 (C-X-C chemokine receptor type 4) receptor and in this way interfere with virus entry into the cell.
The mucosal epithelium of the respiratory track offers another mode of protection from infection. Mucus, a complex mixture of mucins, proteins, proteases, and protease inhibitors, is a major component of the mucosal epithelium. Some bacteria attach to the surface epithelial cells by means of adhesive bacterial surface proteins (eg, the pili of gonococci and Escherichia coli). However, the presence of mucus limits bacterial adhesion to these cell surfaces. Also, once entrapped in the mucus, the bacteria are removed by ciliary clearance. Thus, the mucosal surface and the ciliated epithelial cells tend to inhibit microbial adhesion and limit exposure time. Likewise, the GI track has mechanisms to inhibit bacteria. The acidity of the stomach and the proteolytic enzymes of the small intestine make this environment hostile to many bacteria.
An additional barrier to microbial invasion is the effect of the chemical environment. For example, the presence of an acidic pH in sweat and sebaceous secretions and, as mentioned previously, the low pH of the stomach have antimicrobial properties. Moreover, the production of fatty acids on the skin also tends to eliminate pathogenic organisms.
Although innate immunity does not generate antigen specific protective immunity and does not rely on specific pathogen recognition, it, nevertheless, provides a powerful line of defense. In addition, to the physiologic barriers of protection, the innate system has both cells and proteins (such as, cytokines and complement) at its disposal. Phagocytic leukocytes, such as polymorphonuclear neutrophilic leukocytes (neutrophils), and macrophages along with NK cells are the primary cellular components to combat microbes. The interaction of the invading microbe with these cells and other cells throughout the body triggers the release of complement and numerous cytokines. Many of these cytokines are proinflammatory molecules such as interleukin-1 (IL-1), tumor necrosis factor-alpha (TNF-α), interleukin-6 (IL-6), and the interferons, and are induced through TLR interactions. Armed with these special tools, the host initiates its defense against the invading pathogen.
When a pathogen enters the skin, it is confronted by macrophages and other phagocytic cells possessing “microbial sensors.” There are three major groups of microbial sensors: (1) TLRs, (2) NOD-like receptors (NLRs), and (3) RIG-1–like helicases and MDA-5. The TLRs are the best studied of the microbial sensors. They are a family of evolutionary conserved pattern recognition receptors (PRRs) that recognize pathogen-associated molecular patterns (PAMPs). They constitute a first line of defense against a variety of pathogens and play a critical role in initiating the innate immune response. TLRs are type 1 transmembrane proteins with an extracellular domain, a single transmembrane α-helix, and a cytoplasmic domain. TLR recognition of these specific microbial patterns leads to a signal transduction cascade that generates a rapid and robust inflammatory response marked by cellular activation and cytokine release.
To date, 10 human TLRs have been identified, and each receptor appears to be involved in the recognition of a unique set of microbial patterns. For example, TLR2 recognizes various ligands (eg, lipoteichoic acid) expressed by gram-positive bacteria, whereas TLR3 engages double-stranded RNA (dsRNA) in viral replication. TLR1 and TLR6 recognize multiple diacyl peptides (eg, mycoplasma), whereas TLR4 is specific for gram-negative lipopolysaccharides (LPS). TLR5, on the other hand, recognizes bacterial flagellin, and TLR7 and TLR8 interact with single-stranded RNA (ssRNA) in viral replication and TLR9 binds bacterial and viral DNA. At present, TLR10 remains an orphan receptor.
Another large family of innate receptors, NLRs, are located in the cytoplasm and serve as intracellular sensors for microbial products. They activate the nuclear factor kappa-light chain–enhancer of activated B cells (NF-κB) pathway and drive inflammatory responses similar to the TLRs. The third group of microbial sensors is the RIG-1–like helicases and melanoma differentiation-associated protein 5 (MDA5). These are cytoplasmic sensors of viral ssRNA. The engagement of ssRNA with these sensors triggers the production of the type 1 IFNs. These IFNs are highly effective inhibitors of viral replication.
The key elements of effective innate immunity are responses that are rapid, nonspecific, and of short duration. These features are the hallmark of the phagocytic process. During infection, circulating phagocytic cells increase and can participate in chemotaxis, migration, ingestion, and microbial killing. Any antigen (microorganism) that enters the body through the lymphatics, lung, or bloodstream is engulfed by phagocytic cells.
Therefore, phagocytes, present in the blood, lymphoid tissue, liver, spleen, lung, and other tissues, are the cells responsible for the uptake and removal of foreign antigen. Phagocytes include (1) monocytes and macrophages; (2) granulocytes, including neutrophils, eosinophils, and basophils; and (3) dendritic cells. Monocytes are small leukocytes that circulate in the blood and mature into macrophages that can be found in almost all tissues. For example, they are known as Kupffer cells in the liver and microglial cells in the nervous tissue. Macrophages are critical cells that engulf and kill pathogens, process and present antigen, and regulate immune reactivity by producing a variety of molecules (eg, cytokines).
Granulocytes are leukocytes that contain densely staining granules. Neutrophils have a short half-life and are important phagocytic cells that destroy pathogens within intracellular vesicles. Eosinophils and basophils are less abundant and store granules containing enzymes and toxic proteins that can be released upon activation of the cells. Dendritic cells are also phagocytic and can degrade pathogens; however, their main role is to activate T cells in the adaptive immune response by acting as APCs and by producing regulatory cytokines (eg, IFN-α).
Phagocytosis is a multistep process whereby a phagocytic cell, like a neutrophil, recognizes the pathogen, ingests it, and then destroys the engulfed organism. Once a pathogen enters the blood or tissue, the phagocytic cell migrates to that site. This migration is dependent on the release of chemoattractant signals produced by either the cells of the host or the pathogen. One chemoattractant is IL-8, a potent chemotactic cytokine that attracts neutrophils. More recently IL-17 has been shown to be an effective chemoattractant. In the initial stage of the migration process, neutrophils attach to the endothelial cell surface by means of adhesion molecules, such as P-selectin. Neutrophils follow the chemokine attraction and migrate from the circulation through the endothelium into the tissues and to the site of infection. Here the neutrophil recognizes, engulfs, and internalizes the pathogen into an endocytic vesicle called a phagosome. Once inside the neutrophil, the pathogen is killed.
There are several antimicrobial mechanisms used by phagocytes to eliminate the pathogen. For example, (1) acidification occurs within the phagosome. The phagosome pH is 3.5–4.0, and this level of acidity is bacteriostatic or bactericidal. (2) Toxic oxygen-derived products are generated and include superoxide O2−, hydrogen peroxide H2O2, and singlet oxygen O2. (3) Toxic nitrogen oxides are also produced, and nitric oxide NO is formed. (4) Phagocytic cells generate antimicrobial peptides that participate in pathogen killing. In the macrophage, cathelicidin and macrophage elastase–derived peptides are found. The neutrophil, on the other hand, is rich in α-defensins, β-defensin, cathelicidin, and lactoferricin. All of these mechanisms are used by the phagocytes to destroy the pathogen. When the neutrophil completes its mission, it undergoes apoptosis and dies.
As already mentioned, phagocytosis can occur without antibody. However, phagocytosis is more efficient when antibodies are available to coat the surface of bacteria and facilitate their ingestion. This process is called opsonization and it can occur by the following mechanisms: (1) antibody alone can act as opsonin; (2) antibody and antigen can trigger the complement system (via the classic pathway) to generate opsonin; and (3) opsonin may be produced when the alternative pathway is activated and C3 is generated. Macrophages have receptors on their membranes for the Fc portion of an antibody and for the complement component C3. Both of these receptors facilitate the phagocytosis of the antibody-coated pathogen.
Natural killer (NK) cells are large, granular lymphocytes morphologically related to T cells, which make up 10–15% of blood leukocytes. NK cells contribute to innate immunity by providing protection against viruses and other intracellular pathogens. NK cells have the ability to recognize and kill virus-infected cells and tumor cells. NK cells express two types of surface receptors: (1) lectin-like NK-cell receptors that bind proteins not carbohydrates and (2) killer immunoglobulin-like receptors (KIRs) that recognize the major histocompatibility complex (MHC) class I molecules. These NK-cell receptors have both activation and inhibition properties. NK cells contain large amounts of granzyme and perforin, substances that mediate the cytotoxic actions of NK cells.
In addition, when antibody production is initiated in the adaptive immune response, NK cells play a critical role in antibody-dependent cellular cytotoxicity (ADCC). In this process, specific antibody binds to the target cell surface. The NK cell has Fc receptors that bind to the attached antibody and kill the cell. This property allows the NK cell another opportunity to inhibit the replication of viruses and intracellular bacteria.
NK cells and the IFN system are both integral parts of innate immunity that communicate with each other. NK cells are one of the two primary sources of IFN-γ, a potent antiviral and immunoregulating cytokine. Moreover, the lytic activity of NK cells is enhanced by the type 1 IFNs (IFN-α and IFN-β). These two cytokines are actually induced by the invading virus.
The complement system is another key component of innate immunity. This system consists of 30 proteins found in the serum or on the membrane of selected cells that interact in a cascade. When complement is activated, it initiates a series of biochemical reactions that ultimately culminate in cellular lysis or destruction of the pathogen. As described later in this chapter, there are three complement pathways: classic, alternative, and lectin. Even though each has a different initiating mechanism, they all result in the lysis of the offending invader. The alternative and lectin pathways serve as critical first lines of defense and provide immediate protection against microorganisms. The alternative complement pathway can be activated by microbial surfaces and it can proceed in the absence of antibody. Likewise, the lectin pathway also bypasses antibody and uses a lectin, mannose-binding lectin (MBL), to initiate events. The complement proteins can achieve their defense mission in several ways, including opsonization, lysis of bacteria, and amplification of inflammatory responses through the anaphylatoxins, C5a and C3a. Complement is described in more detail later in this chapter.
Some microbes have acquired mechanisms to sabotage the complement system and evade the immune response. For example, poxviruses, such as vaccinia virus and smallpox, encode a soluble protein with complement regulatory activity that leads to inhibition of the complement system.
In the section on mechanisms of innate immunity, it was mentioned that various cells and complement components of innate immunity orchestrate their effects through the production of soluble mediators. These mediators include cytokines, prostaglandins, and leukotrienes. Here in this section, the role of these mediators in inflammation is outlined. A separate detailed description on cytokines is found in the section on adaptive immune response.
Injury to tissue initiates an inflammatory response. This response is dominated mainly by soluble mediators, referred to as cytokines. Cytokines may include inflammatory and anti-inflammatory cytokines, chemokines, adhesion molecules, and growth factors. During the innate immune response, leukocytes, such as macrophages, release a variety of cytokines, including IL-1 and TNF-α, and IL-6. The other mediators released from activated macrophages and other cells include prostaglandins and leukotrienes. These inflammatory mediators regulate changes in local blood vessels. This begins with dilation of local arterioles and capillaries. During dilation, plasma escapes and accumulates in the area of injury. Fibrin is formed which occludes the lymphatic channels, limiting the spread of organisms.
A second effect of these mediators is to induce changes in the expression of adhesion molecules expressed on the surface of endothelial cells and leukocytes. Adhesion molecules (eg, selectins and integrins) cause leukocytes to attach to the endothelial cells and thereby promote their movement across the vessel wall. Thus, cells stick to the capillary walls and then migrate out (extravasation) of the capillaries in the direction of the irritant. This migration (chemotaxis) is stimulated by proteins in the inflammatory exudate, including some chemokines. A variety of cell types, including macrophages and endothelial cells, can produce chemokines. Once the phagocytic cells migrate to the site of infection, they can initiate the engulfment of microorganisms.
Fever is another common systemic manifestation of the inflammatory response and is a cardinal symptom of infectious disease. The main regulator of body temperature is the thermoregulatory center in the hypothalamus. Among the substances capable of inducing fever (pyrogens) are endotoxins of gram-negative bacteria and cytokines (eg, IL-1, IL-6, TNF-α, and the interferons) released from a variety of cells.
The interferons (IFNs) are critical cytokines that play a key role in defense against virus infections and other intracellular organisms, such as Toxoplasma gondii. Although the IFNs were first identified in 1957 as antiviral proteins, they are now recognized as critical immunoregulating proteins capable of altering various cellular processes, including cell growth, differentiation, gene transcription, and translation. The IFN family consists of three groups. Type I IFNs comprise numerous genes and primarily include IFN-α and IFN-β. Type II IFN consists of a single gene that produces IFN-γ. IFN-λ is a third group of IFN-like cytokines that have more recently been described. Virus infection itself triggers the production of type I IFNs. Following virus entry into a cell, the virus initiates replication and the viral nucleic acid interacts with specific microbial sensors (TLR3, TLR7, TLR 9, RIG-1, and MDA-5). This interaction triggers cellular production of IFN that is secreted from the infected cell. In contrast, the type II IFN, IFN-γ, is produced by activated NK cells in innate immune responses and by specifically sensitized T cells in adaptive immune responses. Moreover, the cytokines IL-2 and IL-12 can trigger T cells to produce IFN-γ.
The IFN system consists of a series of events leading to protection of a cell from virus replication. Once the IFN is produced by the infected cell or the activated NK cell or T cell, the IFN binds to its specific cellular receptor. The IFN receptor interaction activates the JAK, STAT signaling pathways. This process triggers activation of genes that initiate production of selected proteins that inhibit virus replication. All of the IFNs share overlapping biological activities such as antiviral actions, antiproliferative actions, and immunoregulatory actions. However, they also have unique functions that are not overlapping. For example, IFN-β is used successfully to treat patients with multiple sclerosis, whereas IFN-γ has been shown to exacerbate this disease. These potent actions of the IFNs and the advances in biotechnology are the underlying factors that have identified the clinical relevance of the IFNs. In fact, many of the IFNs have been approved by the U.S. Food and Drug Administration (FDA) for the treatment of infections, malignancies, autoimmunity, and immunodeficiency.
ADAPTIVE IMMUNITY
Unlike innate immunity, adaptive immunity is highly specific, has immunologic memory, and can respond rapidly and vigorously to a second antigen exposure. The adaptive immune response involves antibody-mediated and cell-mediated immune responses. An overview of the components and their interactions during the adaptive immune response is outlined as follows, and details are presented throughout this chapter.
Lymphoid cells play a significant role in the adaptive immune response. During embryonic development, blood cell precursors (hematopoietic stem cells) originate in the fetal liver and other tissues; in postnatal life, the stem cells reside in the bone marrow. Stem cells may differentiate into cells of the myeloid or lymphoid series. The lymphoid progenitor cells develop into two main lymphocyte populations: B cells and T cells.
Stem cells destined to become B lymphocytes develop in the bone marrow. They rearrange their immunoglobulin genes and express a unique receptor for antigen on their cell surface. Following this step, they migrate to a secondary lymphoid organ (eg, the spleen) and may be activated by an encounter with antigen to become antibody-secreting plasma cells.
T cells are lymphocytes that are produced in the bone marrow but travel to the thymus to mature. Here, they undergo variable diverse joining (VDJ) recombination of their β chain T cell receptor (TCR) DNA and their α chain TCR DNA. Once TCR rearrangement has occurred and positive and negative selection has terminated, these cells form T cell subclasses with specific functions (eg, CD4 T cells, CD8 T cells). They are the source of cell-mediated immunity.
Figure 8-1 presents a summary of the specific immune processes that are reviewed in this section. The two arms of the immune response, cell-mediated and antibody-mediated, develop concurrently. In the antibody-mediated immune response, CD4 T lymphocytes recognize the pathogen’s antigens bound to the class II MHC molecules on the surface of an antigen-presenting cell (APC) (eg, macrophage, B cell), and as a consequence of this interaction, cytokines are produced that stimulate B cells to express antibodies that display specificity for the antigen. The B cells undergo clonal proliferation and differentiate into plasma cells. In the cell-mediated immune response, the antigen–MHC class II complex is recognized by the CD4 T lymphocyte, whereas the antigen–MHC class I complex is recognized by CD8 T lymphocytes. Both subsets of T cells produce cytokines, become activated, and expand by clonal proliferation. The CD4 T cells that develop stimulate B cells to produce antibodies and promote delayed hypersensitivity while the CD8 T cells direct their activity mainly at the destruction of cells in tissue grafts, tumor cells, or virus-infected cells.
An antigen is a substance that reacts with an antibody. Immunogens induce an immune response and most antigens are also immunogens. There are a wide variety of features that largely determine immunogenicity. They include the following: (1) Recognition of foreignness: Generally, molecules recognized as “self” are not immunogenic. To be immunogenic, molecules must be recognized as foreign (“nonself”). (2) Size: The most potent immunogens are usually large, complex proteins. Molecules with a molecular weight less than 10,000 are weakly immunogenic, and as expected very small molecules are nonimmunogenic. Some small molecules, called haptens, become immunogenic only when linked to a carrier protein. An example is seen with lipids and amino acids that are nonimmunogenic haptens. They require conjunction with a carrier protein or polysaccharide before they can be immunogenic or generate an immune response. (3) Chemical and structural complexity: Chemical complexity is another key feature of immunogenicity. For example, amino acid homopolymers are less immunogenic than heteropolymers that contain two or more different amino acids. (4) Genetic constitution of the host: Because of differences in MHC alleles, two strains of the same species of animal may respond differently to the same antigen. (5) Dosage, route, and timing of antigen administration: Other factors that affect immunogenicity include concentration of antigen administered, route of administration, and timing of antigen administration.
These concepts of immunogenicity are important for designing vaccines in which enhancing immunogenicity is key. However, methods to reduce immunogenicity are also a consideration in protein drug design. This can be seen in an individual who may respond to a certain drug and produce anti-drug antibodies. These anti-drug antibodies may inhibit drug efficacy.
Finally, it should be noted that it is possible to enhance the immunogenicity of a substance by combining it with an adjuvant. Adjuvants are substances that stimulate the immune response by facilitating uptake into APCs.
During the immune response a recognition system capable of distinguishing self from nonself is essential for effective immunity. This section of the chapter concentrates on the molecules used to recognize foreign antigens. Molecules of the MHC and antigen presentation are reviewed first, followed by an overview of the structure and function of antibodies and lastly, an outline of the specific-receptors for antigen recognition (ie, the B-cell receptor [BCR] and the TCR for antigen) is presented.
Historically, the major histocompatibility complex (MHC) was first discovered as a genetic locus that encoded a group of antigens responsible for the rejection of tumor grafts. It is now known that the gene products of this region are the major antigens recognized in transplantation rejection. It is also clear that the MHC molecules bind peptide antigens and present them to T cells. Hence, these molecules are responsible for T-cell antigen recognition and play a significant role in controlling a variety of basic immunologic functions. It should also be noted that the TCR is different from antibody. Antibody molecules bind antigen directly, whereas the TCR only recognizes peptide antigens presented in the context of the MHC molecule on the APC. The TCR is specific for antigen, but the antigen must be presented on a self-MHC molecule. The TCR is also specific for the MHC molecule. Should this antigen be presented by another allelic form of the MHC molecule in vitro, the TCR does not recognize the complex. This is known as MHC restriction.
The MHC is a cluster of well-studied genes closely associated in humans on chromosome 6. The human MHC is called the human leukocyte antigen (HLA) complex. Among the many important genes in the human MHC are those that encode the classes I, II, and III MHC proteins. As outlined in Table 8-1, MHC class I proteins are encoded by the HLA-A, -B, and -C genes. These proteins are made up of two chains: (1) a transmembrane glycoprotein of MW 45,000, non-covalently associated with (2) a non–MHC-encoded polypeptide of MW 12,000 that is known as β2-microglobulin. MHC class I molecules are expressed on nearly all nucleated cells in the body. Key exceptions are observed on cells in the retina and brain.
Class I | Class II | |
---|---|---|
Genetic loci (partial list) | HLA-A, -B, and -C | HLA-DP, -DQ, and -DR |
Polypeptide composition | MW 45,000 + β2M (MW 12,000) | α chain (MW 33,000), β chain (MW 29,000), Ii chain (MW 30,000) |
Cell distribution | Most nucleated somatic cells, except cells of the brain and retina | Antigen-presenting cells (macrophages, dendritic cells, B cells, etc), and IFN-γ–activated cells |
Present peptide antigens to | CD8 T cells | CD4 T cells |
Size of peptide bound | 8–10 residues | 10–30 or more residues |
Class II proteins are encoded by the HLA-D region. The MHC class II proteins consist of three main families: the HLA-DP–, DQ-, and DR-encoded molecules (Table 8-1). This locus controls immune responsiveness and different allelic forms of these genes confer differences in the ability of an individual to mount an immune response.
The HLA-D locus-encoded molecules are cell surface heterodimers that contain two subunits designated α and β that have molecular weights of approximately 33,000 and 29,000 Da, respectively. Unlike class I proteins, the MHC class II proteins have a rather restricted tissue distribution and are constitutively expressed on macrophages, dendritic cells, and B cells. However, the expression of these molecules on other cell types (eg, endothelial cells or epithelial cells) requires induction by IFN-γ.
The MHC class I locus also contains genes that encode proteins required in antigen processing (eg, transporters associated with antigen processing [TAPs] (Figure 8-2). The MHC class III locus encodes complement proteins and several cytokines.
The MHC classes I and II genes exhibit extraordinary genetic variability. Genetic mapping studies showed that there is a high degree of polymorphism in the MHC and different individuals generally express different MHC allelic variants (MHC restriction). It has been noted that over 300 different allelic variants have been defined at some HLA loci. Currently, the MHC genes are the most polymorphic genes known. Each individual inherits a restricted set of alleles from his or her parent. A cluster of tightly linked MHC genes are inherited as a block or haplotype.
In 1987, the three-dimensional structure of the MHC classes I and II proteins was revealed using x-ray crystallography. This elegant work provided critical information on how the MHC proteins function and trigger the immune response. X-ray analysis (Figure 8-3) demonstrates that the entire structure looks like a cleft whose sides are formed by the α helices and whose floor is shaped by the β-pleated sheets. The x-ray analysis also shows that the cleft is occupied by a peptide. In essence, the TCR sees the peptide antigen bound in a cleft provided by the MHC protein. Figure 8-4A illustrates this interaction.
FIGURE 8-4
Binding of antigen by MHC and T-cell receptor (TCR). In panel A, a model of the interaction between peptide antigen, MHC, and the TCR is shown. The Vα and Vβ regions of the TCR are shown interacting with the α helices that form the peptide-binding groove of MHC. In panel B, a model of the interaction between a superantigen, MHC, and the TCR, is shown. The superantigen interacts with the Vβ region of the TCR and with class II MHC outside the peptide-binding groove. (Adapted with permission from Stites DG, et al [editors]: Medical Immunology, 9th ed. McGraw-Hill, 1997. © The McGraw-Hill Companies, Inc.)
The MHC proteins display broad specificity for peptide antigens. In fact, many different peptides can be presented by a different MHC allele. A key to this model is that the MHC polymorphism allows for the binding of many specific and different peptides in the cleft. This means that different alleles can bind and present different peptide antigens.
Antigen processing and presentation represent the hallmark of the adaptive immune response. This complex mechanism of antigen recognition begins with antigens that become associated with self-MHC molecules for presentation to T cells with appropriate receptors. Proteins from exogenous antigens, such as bacteria, are internalized by the APC (dendritic cells or macrophages) and undergo denaturation or partial proteolysis in the endocytic vesicles within the APC. While in the endosomal compartment, these peptide fragments fuse with exocytic vesicles containing MHC class II molecules. As noted in Figure 8-2, this step exposes the appropriate linear peptide fragment that eventually becomes expressed on the surface of the APC (as the peptide-MHC complex).
The MHC class II molecules are synthesized in the rough endoplasmic reticulum (ER) and then they proceed out through the Golgi apparatus. The invariant chain, a polypeptide that helps transport the MHC molecules, complexes with the MHC class II complex in an endosome. This vesicle is called the MHC class II compartment. This invariant chain is useful and blocks the binding of self-endogenous cellular peptides into the MHC class II complex. The invariant chain is now enzymatically removed. Through a series of steps, the MHC class II binds exogenous antigen (peptide fragments) and is transported to the cell membrane for presentation.
The interaction of endogenous antigens within a virus-infected cell and the MHC class I molecule is outlined in Figure 8-2. In brief, cytosolic proteins are broken down by a proteolytic complex called the proteasome. The cytosolic peptides gain access to nascent MHC class I molecules in the rough ER via the peptide transporter systems (TAPs). The TAP genes are also encoded in the MHC. Within the lumen of the ER, peptide antigens approximately 8–10 residues in length complex with nascent MHC class I proteins and cooperate with β2-microglobulin to create a stable, fully folded MHC class I–peptide antigen complex that is then transported to the cell surface for display and recognition by CD8 cytotoxic T cells. The binding groove of the class I molecule is more constrained than that of the class II molecule, and therefore, shorter peptides are found in class I than in class II MHC molecules. Once the cytotoxic T cell recognizes the MHC class I peptide antigen, it can now kill the virus-infected cell.
Several viruses attempt to defeat the immune response by interfering with the antigen-processing pathways. For example, an HIV Tat protein is able to inhibit expression of class I MHC molecules. A herpesvirus protein binds to the TAPs, preventing transport of viral peptides into the ER, where class I molecules are being synthesized. A consequence of these inhibitory mechanisms is that the infected cells are not recognized by cytotoxic lymphocytes.
Some bacterial and viral antigens are able to activate large numbers of T cells through a special pathway. These proteins are called superantigens. Superantigens do not require processing and therefore are able to bind to MHC molecules outside the peptide-binding cleft (Figure 8-4B). Compared to the standard antigen-induced T-cell response where a small number of T cells are activated, superantigens can stimulate much larger numbers (~25% more) of the T cells. Classic examples of superantigens include certain bacterial toxins, including the staphylococcal enterotoxins, toxic shock syndrome toxin, and group A streptococcal pyrogenic exotoxin A. A consequence of this massive activation of T cells is the overproduction of cytokines, in particular, IFN-γ. IFN-γ in turn activates macrophages to produce IL-1, IL-6, and TNF-α, all which may contribute to a “cytokine storm” causing severe symptoms of shock and multiple organ failure.
Humoral immunity is mediated by antibodies. Each individual has a large pool of unique B lymphocytes (~1011) that have a life span of days or weeks and are found in the blood, lymph, bone marrow, lymph nodes, and gut-associated lymphoid tissues (eg, tonsils, Peyer patches, appendix).
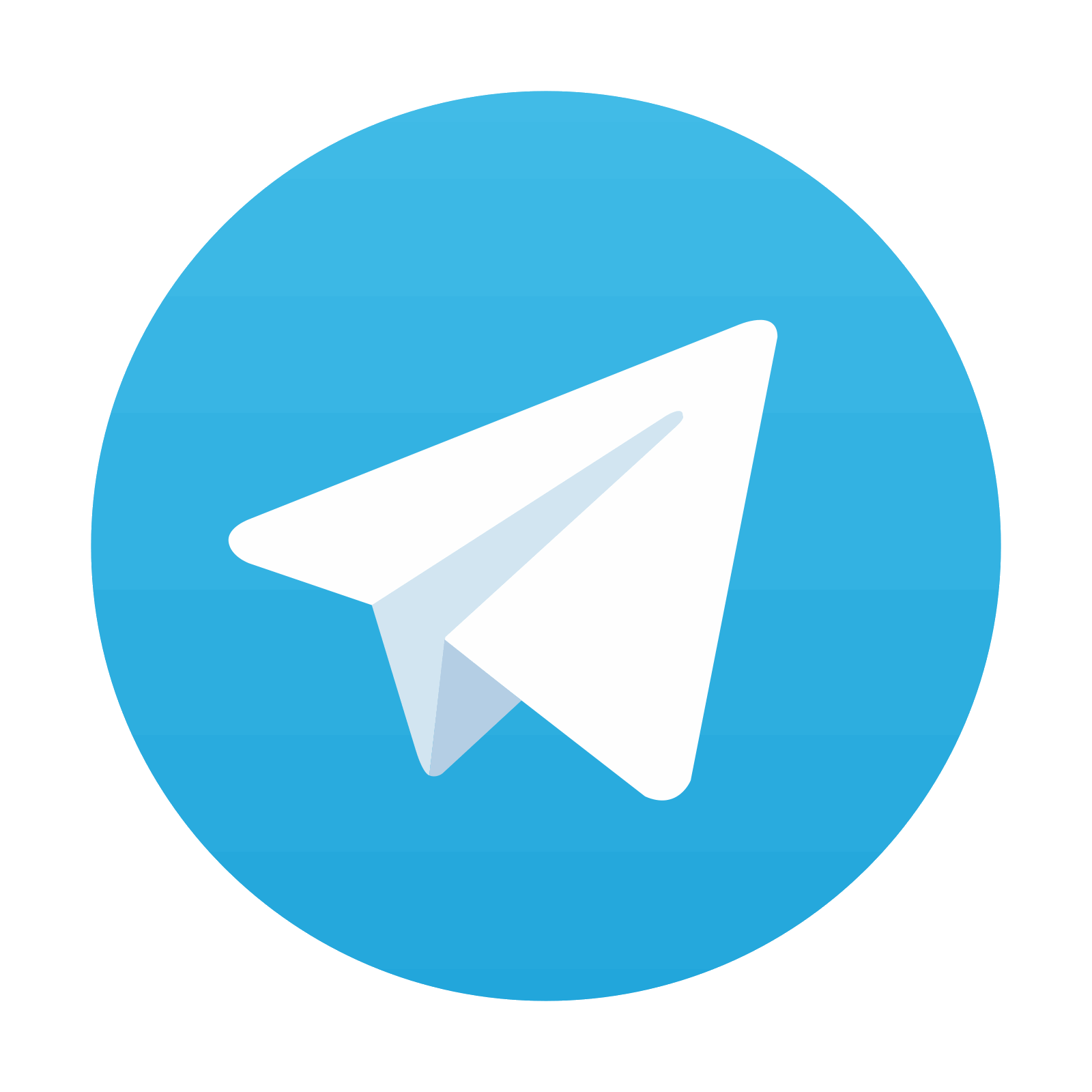
Stay updated, free articles. Join our Telegram channel
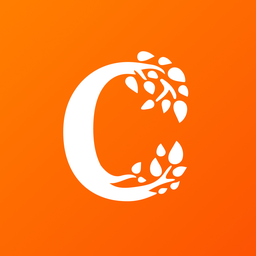
Full access? Get Clinical Tree
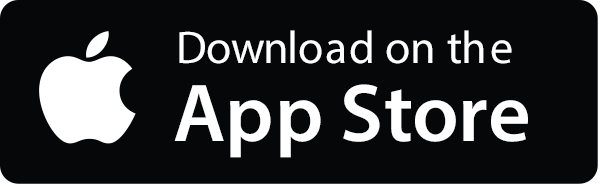
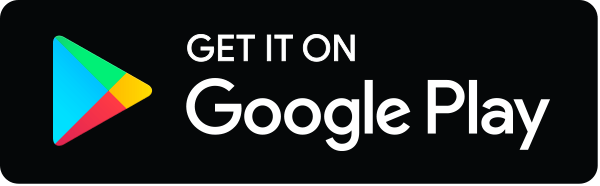