Hypercoagulable States
DEFINITION
The concept of a state of hypercoagulability dates back to 1854, when German pathologist Rudolph Virchow postulated that thrombosis resulted from, and in turn precipitated, three interrelated factors1: decreased blood flow (venous stasis), inflammation of or near the blood vessels (vascular endothelial injury), and intrinsic alterations in the nature of the blood itself. These “blood changes” alluded to in Virchow’s triad have become what are now known as hypercoagulable states, or thrombophilias.
Most hypercoagulable states alter the blood itself, whereas others affect the vasculature directly. Although patients with hypercoagulable states are at greater risk for developing a thrombotic event than those without such disorders, not all persons with a well-defined hypercoagulable state will develop an overt thrombosis and not all persons with thrombosis have an identifiable hypercoagulable state. In fact, in 2003, testing for an inherited hypercoagulable state was likely to uncover an abnormality in more than 60% of patients presenting with idiopathic (i.e., spontaneous or unprovoked) VTEs (Fig. 1).2 Although the remaining 30% to 40% would have unremarkable test results, this does not imply a true absence of a hypercoagulable state. Some of these individuals may have an acquired condition such as cancer or APA, and others may have a disorder or defect that has not yet been discovered or characterized. This can be illustrated by the fact that before 1993—before the discoveries of factor V Leiden and the prothrombin G20210A mutation—an inherited predisposition to hypercoagulability was identified in only 15% to 20% of patients presenting with idiopathic VTEs.2
This chapter will focus on the most common hypercoagulable states and their association with VTEs. Readers are referred to reports on the association between hypercoagulable states and arterial thrombosis.3,4 Specific derangements that will be reviewed in this chapter include activated protein C resistance, factor V Leiden, prothrombin G20210A mutation, deficiencies of natural anticoagulants (antithrombin, protein C, and protein S), APAs, and hyperhomocysteinemia. Although it is beyond the scope of this chapter to review the association between cancer and thrombosis or to discuss issues pertaining to cancer screening following VTEs, malignant diseases are likely the most common acquired hypercoagulable state. At a minimum, appropriate age- and gender-specific cancer screening must be considered after VTEs, particularly in older individuals and in young patients with idiopathic VTEs but without laboratory evidence for a hypercoagulable state. Guidelines for the early detection of cancer have been updated by the American Cancer Society.5
ETIOLOGY
Factor V Leiden, or factor V G1691A, is a single-point mutation in the gene that codes for coagulation factor V.2 It involves a G (guanine)-to-A (adenine) substitution at nucleotide 1691 (G1691A) in exon 10, which predicts the replacement of arginine at amino acid residue 506 by glutamine (Arg506Gln).6 The mutation, transmitted through autosomal dominant inheritance, renders factor V resistant to inactivation by APC (a natural anticoagulant protein). Factor V Leiden accounts for 92% of cases of APC resistance (APC-R), with the remaining 8% of cases resulting from pregnancy, oral contraceptive use, cancer, select APA, and other factor V point mutations. Therefore, the terms factor V Leiden and APC-R should not be considered synonymous; in fact, APC-R is an independent risk factor for VTEs, even in the absence of factor V Leiden. It is estimated that the mutation arose in a single white ancestor some 21,000 to 34,000 years ago, well after the evolutionary separation of non-Africans from Africans (approximately 100,000 years ago) and of Caucasoid (white non-Africans) from Mongoloid (Asians) subpopulations (approximately 60,000 years ago).7
Prothrombin G20210A is a single-point mutation (G-to-A substitution at nucleotide 20210) in the 3′ untranslated region of the prothrombin (coagulation factor II) gene.2 This autosomal dominant mutation appears to result in elevated concentrations of plasma prothrombin. Like factor V Leiden, the prothrombin G20210A mutation arose in a single common white founder, and probably also occurred after the evolutionary divergences of subpopulations.8
Over 100 different mutations have been detected in the genes that code for each of the natural anticoagulant proteins (protein C, protein S, and antithrombin), resulting in quantitative (type I) or qualitative (type II) deficiencies.9 Both antithrombin and protein S deficiencies have an autosomal dominant pattern of inheritance.10,11 Protein C deficiency was believed to be inherited in an autosomal dominant pattern, with incomplete penetrance. Studies have suggested that protein C deficiency is an autosomal recessive disorder and that coinheritance of another defect, particularly factor V Leiden, results in a high degree of penetrance that appears as dominant inheritance in double-heterozygous carriers.12,13
APAs consist of two major subgroups, the lupus anticoagulants and the anticardiolipin antibodies. Lupus anticoagulants are detected by their ability to prolong phospholipid-dependent coagulation tests in vitro (e.g., activated partial thromboplastin time and dilute Russell viper venom time). Anticardiolipin antibodies are detected by the enzyme-linked immunosorbent assay (ELISA).14 APAs that occur in association with autoimmune disorders, such as systemic lupus erythematosus, Sjögren’s syndrome, rheumatoid arthritis, and mixed connective tissue disease, as well as in the setting of cancer, are considered secondary;15 APAs detected in those without any obvious underlying autoimmune or malignant diseases are called primary. APAs have also been reported in conjunction with idiopathic autoimmune hemolytic anemia, malaria, syphilis, Q fever, infections by mycobacteria, Pneumocystis jiroveci, cytomegalovirus, and after exposure to drugs such as neuroleptics, quinidine, and procainamide.14,16
The metabolism of the amino acid homocysteine consists of a vitamin B6-dependent trans-sulfuration pathway involving the enzyme cystathionine β-synthase (CBS) and a folate- and vitamin B12–dependent remethylation pathway involving the enzymes methylenetetrahydrofolate reductase (MTHFR) and methionine synthase (Fig. 2).17,18 Inherited severe hyperhomocysteinemia (plasma level >100 µmol/L), as seen in classic homocystinuria, may result from homozygous MTHFR or CBS deficiencies and, more rarely, from inherited errors of cobalamin (vitamin B12) metabolism.19 Inherited mild to moderate hyperhomocysteinemia (plasma level 15-100 µmol/L) may result from heterozygous MTHFR and CBS deficiencies, but most commonly results from the C677T gene polymorphism, which is the most common mutation in the gene that codes for the MTHFR enzyme.18,19 This single-point mutation (C677T) in the coding region for the MTHFR binding site (exon 4) is autosomal recessive, leads to the substitution of a valine for an alanine, and results in a thermolabile variant of the MTHFR (tlMTHFR).18 Acquired hyperhomocysteinemia in the absence of any mutation or polymorphism may be caused by folate deficiency, vitamins B6 and B12 deficiencies, renal failure, diabetes mellitus, hypothyroidism, carcinoma, pernicious anemia, inflammatory bowel disease, and methotrexate, theophylline, or phenytoin therapy.17,18
Individuals who are heterozygous for the tlMTHFR variant have normal plasma homocysteine levels, whereas homozygous carriers may have mild to moderate fasting hyperhomocysteinemia in the setting of concomitant folate deficiency.17,19 However, homozygosity for the tlMTHFR in the absence of hyperhomocysteinemia does not appear to be associated with an increased risk of VTEs, and most patients with hyperhomocysteinemia do not have the tlMTHFR polymorphism.19 Excess homocysteine in the plasma is the risk factor and is the target of therapeutic intervention, not the C677T mutation.
PREVALENCE
The prevalence of hypercoagulable states depends on the ethnicity and clinical history of the studied population. Prevalence is lowest in the general population, greater in individuals with a single VTE, and highest in those with recurrent VTEs or who are from known thrombophilic families (Table 1).20
APC-R caused by factor V Leiden is the most common inherited predisposition to hypercoagulability in white populations of northern European background.2 Factor V Leiden follows a geographic and ethnic distribution; it occurs most frequently in northern and western Europe (the highest prevalence of 15% has been reported in Sweden), but high prevalences are also found in Cyprus (13%), Turkey (9%), and the Middle East (5.4%).21 The mutation is also found in whites from eastern Europe and South America (prevalences of 2%-4%), but is rare in the Asian and African continents as well as in ethnic groups of Asian descent, such as the Inuit Eskimos, Native Americans, Australian aboriginals, and Polynesians.21 In the United States, factor V Leiden is most commonly seen in whites (6%), with lower prevalences in Latin Americans (2.2%), African Americans and Native Americans (1.2%), and Asian Americans (0.45%).22
The prothrombin G20210A mutation is the second most common inherited predisposition to hypercoagulability, occurring more frequently in whites of southern European background. In fact, the 3% prevalence in southern Europe is almost twice the prevalence observed in northern Europe.23 Similar to factor V Leiden, the prothrombin G20210A mutation is also found in the Middle East and Indian regions, but is virtually absent in individuals of African and eastern Asian backgrounds.23 These distributions provide support to the estimate that both mutations (factor V Leiden and prothrombin G20210A) originated relatively recently in the European founding population, after the evolutionary divergences of subpopulations.
The deficiencies of natural anticoagulants are rare in the general population and, combined, are found in less than 15% of all individuals with a single VTE (see Table 1 and Fig. 1). The prevalence of APA (an acquired set of disorders) is significantly higher in patients with autoimmune disorders than in healthy individuals from the general population. In patients with systemic lupus erythematosus, the reported average prevalences for lupus anticoagulants and anticardiolipin antibodies are 34% and 44%, respectively.15
PATHOPHYSIOLOGY
Secondary hemostasis consists of a series of sequential reactions, a coagulation cascade, in which inactive protease zymogens are converted to active serine proteases, ultimately resulting in the production of thrombin and covalently cross-linked fibrin (Fig. 3). In response to vascular injury, the in vivo coagulation cascade is triggered by the exposure of tissue factor. Tissue factor not only complexes with trace amounts of activated factor VII, present in the circulation of normal individuals, but also activates factor VII to factor VIIa. The complex formed by factor VIIa and tissue factor then activates factors IX and X, leading to the formation of small amounts of thrombin. However, this pathway is rapidly downregulated by tissue factor pathway inhibitor. Nevertheless, potent positive feedback by thrombin itself results in the activation of factor XI to factor XIa, which can activate factor IX, hence perpetuating the production of thrombin and, subsequently, of a fibrin clot. Thrombin also promotes ongoing thrombosis by activation of factors VIII, V, and XIII. Factor VIIIa functions as a cofactor during the activation of factor X to Xa, catalyzed by factor IXa. Factor Va functions as a cofactor during the activation of prothrombin to thrombin, catalyzed by factor Xa. The end result of these sequential reactions is the conversion of fibrinogen to fibrin monomers. Factor XIIIa cross-links fibrin to promote the development of a stabilized platelet plug (see Fig. 3).

Figure 3 Coagulation cascade.
PT, prothrombin time; TF, tissue factor; TFPI, tissue factor pathway inhibitor; XL, cross-linked.
The natural anticoagulants function to confine thrombus formation to the site of vascular injury and to limit thrombus size. Whereas it promotes ongoing coagulation by a number of positive feedbacks, thrombin also provides an important negative feedback to limit thrombus formation by binding to thrombomodulin on endothelial cells. The thrombin-thrombomodulin complex then converts protein C to APC. Antithrombin and protein C are the major natural anticoagulants, and protein S serves as a vital cofactor for APC-mediated inactivation of factors Va and VIIIa (see Fig. 3).
Vascular endothelial disruption triggers not only coagulation reactions but also the fibrinolytic pathways (Fig. 4). Physiologic fibrinolysis is initiated by endothelial cell-derived tissue plasminogen activator (tPA)-mediated conversion of plasminogen to plasmin. Plasmin can degrade fibrinogen and fibrin, thus limiting the size of a thrombus and helping to clear a thrombus once the vascular injury has been repaired. The fibrinolytic pathways are regulated by the inhibitory proteins α2-antiplasmin and plasminogen activator inhibitor-1.
Therefore, the human hemostatic system can be defined as consisting of multiple independent yet integrally related cellular and protein components; these function to maintain blood fluidity under normal conditions and to promote localized, temporary thrombus formation at sites of vascular injury (Fig. 5). The six major components of this hemostatic system are vascular endothelium, platelets, plasma coagulation proteins or “factors,” natural anticoagulant proteins, fibrinolytic proteins, and antifibrinolytic proteins. In the presence of an intact endothelium, there is no clot formation taking place inside the blood vessels, even though a low, basal, physiologic level of coagulation factor activation is occurring continuously. This highly regulated hemostatic system maintains a delicate balance between a prohemorrhagic and prothrombotic state, which is maintained by the concomitant actions of platelets, coagulation factors, and fibrinolytic inhibitors (on one side of the hemostatic scale), and of natural anticoagulants and fibrinolytic proteins (on the other side of the scale), as shown in Figure 6. Marked thrombocytosis, accentuated platelet aggregation, increased activity levels of coagulation factors, and excess plasma levels of fibrinolytic inhibitors may lead to pathologic thrombosis. Similarly, quantitative or qualitative deficiencies of a natural anticoagulant coagulation factor resistance to inactivation by a natural anticoagulant (in the specific case of factor V Leiden), and a deficiency of a fibrinolytic protein, may all be associated with a state of hypercoagulability. Thus, it is not surprising that a multitude of potential hypercoagulable states have been described (Box 1).
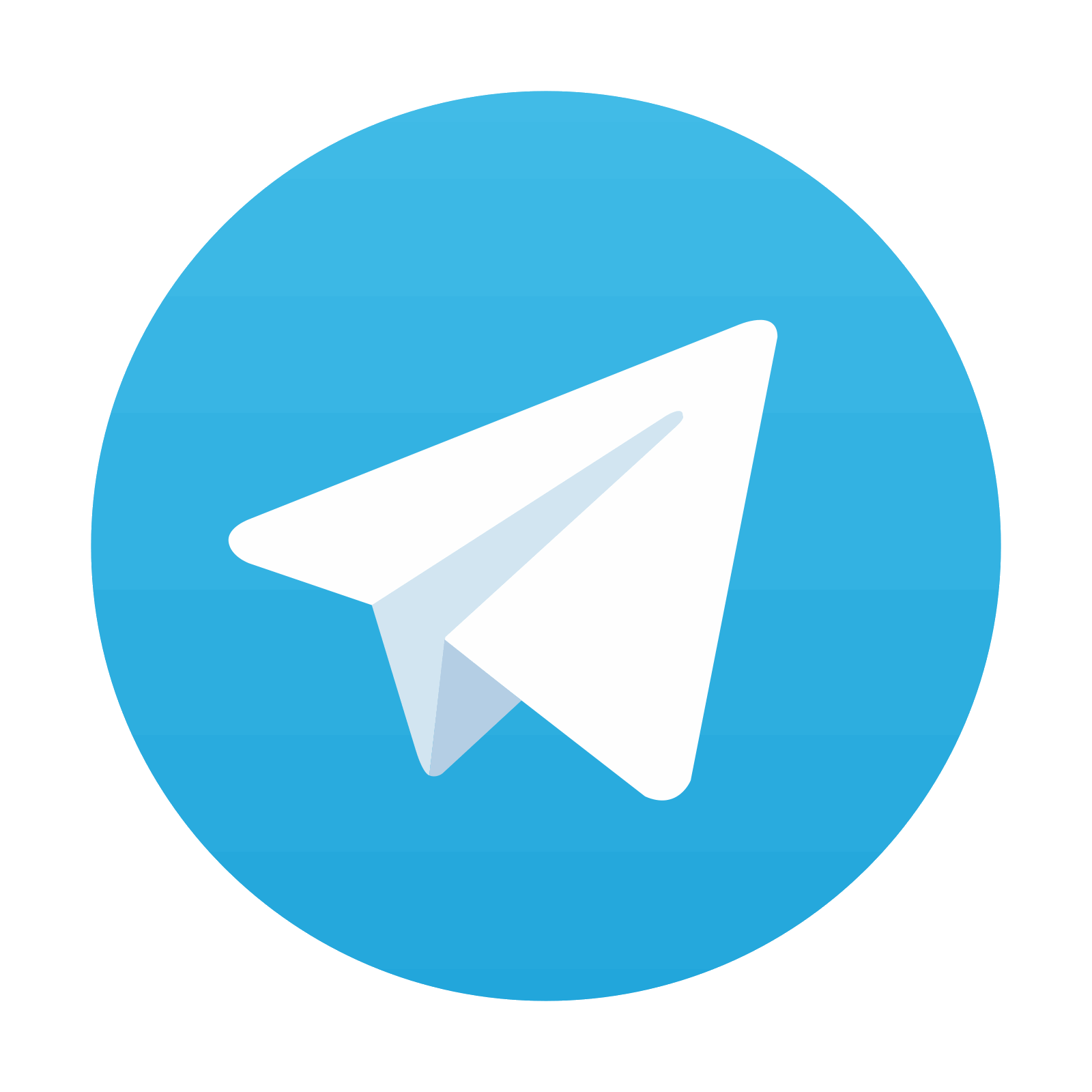
Stay updated, free articles. Join our Telegram channel
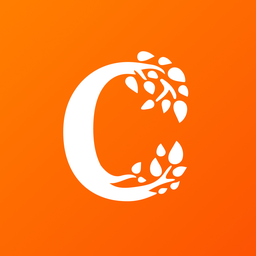
Full access? Get Clinical Tree
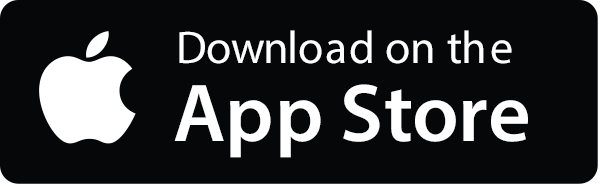
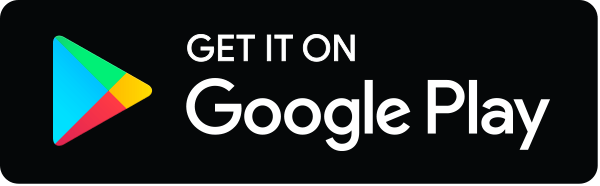