Hematopoietic Stem Cell Transplantation
KEY CONCEPTS
Hematopoietic stem cell transplantation (HSCT) is a process that involves IV infusion of hematopoietic stem cells from a donor into a recipient, after the administration of chemotherapy with or without radiation. The rationale is to increase tumor cell kill by increasing the dose of chemotherapy. Immune-mediated effects also contribute to the tumor cell kill observed after allogeneic HSCT.
Hematopoietic stem cells used for transplantation can come from the recipient (autologous) or from a related or unrelated donor (allogeneic). If the related donor is a twin, the transplant is referred to as a syngeneic transplant.
Human leukocyte antigen (HLA) mismatching of allogeneic donor–recipient pairs at either class I or class II loci increases the risk of graft failure, graft-versus-host disease (GVHD), and worse survival. The ideal donor is one that is matched at HLA-A, -B, -C, and DRB1.
Hematopoietic stem cells are found in the bone marrow, peripheral blood, and umbilical cord blood. Because of the rarity and similarity to other cells, hematopoietic stem cells are difficult to isolate and measure. These stem cells express the CD34 antigen, and measurement of the number of CD34+ cells is a clinically useful measure of the number of hematopoietic stem cells.
Because of clinical and economic advantages, peripheral blood has replaced bone marrow as the source of hematopoietic stem cells in the autologous and adult allogeneic HSCT setting.
The purpose of the preparative (or conditioning) regimen in traditional myeloablative transplants is twofold: (a) maximal tumor cell kill and (b) immunosuppression of the recipient to reduce the risk of graft rejection (allogeneic HSCT only).
Reduced-intensity conditioning regimens (including those that are nonmyeloablative) have been developed in order to reduce early posttranslant morbidity and mortality while maximizing the GVT effect of the allogeneic graft. The advantage to this approach is that patients who would otherwise not be eligible for allogeneic HSCT can now be offered a potentially curative therapy.
The transplant-related mortality rate associated with allogeneic HSCT ranges from 10% to 80% depending mostly on age and donor and disease status. Major causes of death include infection, organ toxicity, and GVHD. The most common cause of death after autologous HSCT is disease relapse; the transplant-related mortality rate is usually less than 5%, depending on the conditioning regimen, age, and disease status.
Patients undergoing allogeneic HSCT are given prophylactic immunosuppressive therapy, which inhibits T-cell activation, proliferation, or both. The most commonly used GVHD prophylaxis regimens are cyclosporine or tacrolimus and methotrexate.
Initial treatment of both acute and chronic GVHD consists of prednisone, either alone or combined with cyclosporine or tacrolimus. Treatment of patients with steroid-refractory GVHD is unsatisfactory.
INTRODUCTION
Hematopoietic stem cell transplantation (HSCT) is a process that involves IV infusion of hematopoietic stem cells from a compatible donor into a recipient, usually after administration of high-dose chemotherapy with or without radiation (called conditioning or preparative regimens). The original rationale for HSCT for treatment of malignant disease is based on studies showing that most anticancer drugs have a steep dose–response relationship and that myelosuppression limits the chemotherapy dosage that can be safely administered. Although standard-dose chemotherapy can prolong survival in many cancer patients, most patients are not cured of their disease with this strategy alone. Infusion of hematopoietic stem cells allows administration of very high doses of chemotherapy (as much as 10-fold higher) by reestablishing hematopoiesis. If tumor cells that are resistant to standard doses are sensitive to higher doses of chemotherapy, then tumor cell kill will be greatly increased, and the likelihood of cure would be higher with HSCT compared with standard dose chemotherapy. However, the chemotherapy dose cannot be escalated indefinitely because of the risk for death caused by nonhematologic toxicity (Fig. 117-1). The success of reduced-intensity conditioning (RIC) regimens shows that immune-mediated effects of the donor cells also contribute to the antitumor effect of allogeneic HSCT.
FIGURE 117-1 Patients represented by the middle column are the best candidates for hematopoietic stem cell transplantation because the technique allows for administration of chemotherapy or radiation in doses that otherwise would be intolerable because of severe myelosuppression.
HSCT is an important modality for treatment of a variety of malignant and nonmalignant diseases. More than 16,000 transplants were performed in the United States in 2009, primarily for malignant diseases.1 The most common malignancies treated with HSCT are multiple myeloma, lymphoma, and leukemia. The number of transplants has grown steadily over the past decade because of an increase in the number of patients receiving umbilical cord blood (UCB) transplants and patients older than 60 years undergoing transplantation.
Although HSCT is most commonly used for treatment of malignant diseases, many nonmalignant hematologic disorders, including aplastic anemia, thalassemia, and sickle cell anemia; immunodeficiency disorders; and other genetic disorders are also potentially curable with allogeneic HSCT. Transplantation is also being investigated as a treatment modality for patients with life-threatening autoimmune diseases, such as rheumatoid arthritis, systemic and multiple sclerosis, and systemic lupus erythematosus.
This chapter summarizes the procedures involved in HSCT and the common complications associated with HSCT. More detailed information on HSCT can be found in published reviews and books.2–5 Information on HSCT also can be found on several websites, including http://www.cibmtr.org (Center for International Blood and Marrow Transplant Research [CIBMTR]) and http://www.marrow.org (National Marrow Donor Program).
DONORS AND HISTOCOMPATIBILITY TESTING
Different types of donors are used in HSCT. The choice of donor depends on the diagnosis and disease status of the recipient as well as his or her age and comorbidities. The role and indications for HSCT are discussed in detail within individual disease chapters of this text. In autologous transplants, patients receive their own hematopoietic stem cells, which were collected and stored before administration of the transplant conditioning regimen. In syngeneic transplants, an identical twin serves as the donor. In allogeneic transplants, the donor is genetically not identical to the recipient but shares some common cell surface antigens called human leukocyte antigens (HLAs). These antigens are encoded by the major histocompatibility complex (MHC), a cluster of genes located on the sixth chromosome.6 The MHC contains three distinct regions designated as class I, class II, and class III. Class I and class II genes encode for HLA; products of class III genes have other important roles in the immune system. Class I and class II HLA antigens differ in their tissue distribution, structure, and function. Their primary function is to aid the immune system in recognizing cells or tissues as “self” or “nonself.” The genes (and the corresponding antigens they encode for) important in HSCT are HLA-A, HLA-B, and HLA-C (class I) and HLA-DRB1 (class II). Because of the polymorphism of the HLA system, there are many different HLA antigens within each different class of HLA. To reduce the chance of graft rejection and graft-versus-host disease (GVHD), a donor is chosen based on how many of these HLA antigens are the same as those of the recipient.
To identify a suitable allogeneic donor, both the recipient and potential donors are HLA typed (specific HLA antigens are identified); the potential donor who is most closely matched (has the most similar HLA antigens to the recipient) is generally chosen to be the transplant donor. HLA typing is accomplished by DNA-based techniques that use polymerase chain reaction (PCR) amplification of specific HLA genes from genomic DNA. DNA typing methods are categorized by the level of discrimination they provide in defining the sequence of an HLA gene. Low-resolution methods provide limited sequence information about a particular HLA gene and are typically used to identify sibling donors. However, low-resolution techniques cannot distinguish the extremely polymorphic nature of many of the HLA antigens. HLA antigens are characterized by thousands of genetic variations (alleles), and each allele may correspond to a unique HLA molecule. Different alleles can be distinguished only by high-resolution typing techniques; high-resolution methods are used to identify suitable unrelated donors.
The degree of HLA mismatching correlates with the risk of graft rejection, GVHD, and survival.6 In an analysis of 1,874 patients who received HLA-matched unrelated bone marrow donor transplants under the auspices of the National Marrow Donor Program (NMDP), low-resolution mismatches at HLA-A, HLA-B, HLA-C, and HLA-DRB1 were similarly associated with increased risk of GVHD and mortality.7 The observation concerning the prognostic value of HLA-C was particularly important because until that time, the locus was omitted from most matching algorithms. Based on these results, HLA-C typing is now included in standard typing protocols. High-resolution mismatches, particularly at HLA-A and HLA-DRB1, also were associated with increased mortality. These findings were confirmed in an analysis of more than 3,800 unrelated bone marrow donor–recipient pairs in which high-resolution matching for HLA-A, -B, -C, and DRB1 was associated with the overall survival.8
In the search for an allogeneic donor, the patient’s siblings are typed first. The odds that any one full sibling will match a patient are one in four. About 30% of Americans have an HLA-identical sibling. In an effort to offer allogeneic HSCT to patients who lack an HLA-identical sibling donor, alternative donors are being used. Rarely, a parent is HLA identical with his or her child. Although some patients who receive transplants from mismatched related donors experience long-term survival, their risks of graft failure and acute GVHD are higher than for recipients of matched-sibling transplants. It is estimated that only another 10% of patients will have a closely HLA-matched related donor.
The most common type of alternative donor is an individual unrelated to the recipient who is fully or closely HLA matched. To facilitate identification of these donors, the NMDP (http://www.marrow.org) was started in 1986 with initial funding from a U.S. Navy contract. To date, the NMDP has registered more than 10 million donors in the United States and has facilitated more than 50,000 unrelated donor transplants. Donors outside the United States can also be accessed by the NMDP through agreements with international cooperative registries. About one-third of the allogeneic HSCTs performed worldwide are from unrelated donors.1 The NMDP currently requires that the recipient be typed by high-resolution methodology at HLA-A, -B, -C, and -DRB1. Although it is the transplant center’s responsibility to select the donor, the NMDP recommends that selected donor and recipient be matched at HLA-A, -B, -C, and -DRB1 by high-resolution typing when possible for bone marrow or peripheral blood HSCT; matching criteria are less stringent for UCB transplant.9 If more than one suitable HLA-matched unrelated donor is identified, other factors can be used to select the donor, such as younger age, being male or a nulliparous female, and negative cytomegalovirus (CMV) serostatus.
The likelihood of a recipient finding an HLA-matched donor ranges from one in 100 to one in 1,000,000, depending on the prevalence of the recipient’s HLA type, race, and ethnic background. With the current size of the NMDP registry, the matching likelihood is higher than 80% for whites. Because most minorities are not as well represented in the program, the likelihood of finding a donor for patients from some racial or ethnic groups is lower. Agreements between NMDP and international registries may improve the likelihood of finding donors for these patients. Another limitation is the time needed to search for a potential donor. Some donor searches take up to 3 to 4 months, and patients with acute leukemia can relapse while waiting for completion of the search. Cost is also a concern, with the cost for donor search and procurement ranging from $25,000 to $50,000. With improved HLA typing techniques and better supportive care, most reported outcomes with matched unrelated donors are no longer significantly different than those reported with related sibling donors.10,11
HEMATOPOIETIC STEM CELLS
Hematopoietic stem cells serve as “mother” cells for all blood cells, including erythrocytes, leukocytes, and platelets (see eChap. 20). Stem cells have varying degrees of “stemness.” True pluripotent stem cells are capable of replicating indefinitely and can give rise to stem and progenitor cells of all tissues. Multipotent stem cells, such as hematopoietic stem cells, have the capacity for self-renewal and can differentiate into more than one cell type in a particular tissue lineage. Because of their capacity for self-renewal, hematopoietic stem cells are capable of repopulating the recipient’s marrow, which has been “emptied” by administration of high-dose chemotherapy, either alone or combined with radiation.
Hematopoietic stem cells are rare cells, comprising less than 0.01% of all bone marrow cells. Isolation and quantitative measurement of hematopoietic stem cells are extremely difficult because of their rarity and their similar appearance to other cells. For these reasons, surrogate markers are used to measure the number of stem cells. CD34 is an antigen expressed on hematopoietic stem cells and other early progenitor cells. Determination of the number of cells expressing the CD34 antigen (CD34+ cells), as determined by flow cytometry, has become the standard method of measuring hematopoietic stem cell content.
Hematopoietic stem cells are found in the bone marrow, peripheral blood, and UCB. Hematopoietic stem cells from the bone marrow are obtained by multiple aspirations from the anterior and posterior iliac crests while the donor is under general anesthesia. The procedure takes about 1 hour and yields 200 to 1,500 mL, depending on the size of the donor. The marrow is transferred into tissue culture medium containing preservative-free heparin. The pooled marrow is passed through a series of stainless steel screens to break up aggregated particles, resulting in an essentially single-cell suspension. In allogeneic HSCT, the marrow stem cells are given to the recipient 12 to 24 hours after harvest. In autologous HSCT, the marrow is frozen and stored until needed. After IV infusion, the marrow stem cells enter the systemic circulation and find their way to the bone marrow cavity, where they reseed and grow in the bone marrow microenvironment. Although the donor experiences local soreness for a few days, the procedure usually is well tolerated, with no delayed complications resulting from the marrow aspiration. The major risk of serving as a marrow donor is the risk of undergoing general anesthesia.
Hematopoietic stem cells in peripheral blood (peripheral blood stem cells [PBSCs]) are found in the mononuclear fraction of white blood cells (lymphocytes and monocytes) and are collected by a procedure called leukapheresis (or apheresis). This is an outpatient procedure that involves withdrawal of blood from a vein (through a specialized IV catheter), selective removal of mononuclear cells by an apheresis machine, and reinfusion of the unneeded blood components back to the patient. During this process, about 9 to 14 L of blood is processed over several hours during each daily apheresis session. Most of the blood cells are returned to the donor, and each apheresis yields about 200 mL of cells.
The number of hematopoietic stem cells that circulate in peripheral blood normally is too low for apheresis to be technically feasible. Without mobilization techniques, at least six aphereses usually are required to collect a sufficient number of PBSCs. Several methods have been used clinically to “mobilize” hematopoietic stem cells from the bone marrow into peripheral blood for use in autologous transplantation.12 Figure 117-2 shows representative schemas for mobilization and collection of PBSCs. One type of mobilization method is administration of chemotherapy, which can briefly increase the number of PBSCs as much as 100-fold. The more commonly used method is administration of a recombinant hematopoietic growth factor such as granulocyte colony-stimulating factor (G-CSF; filgrastim) or granulocyte-macrophage colony-stimulating factor (GM-CSF; sargramostim). Each agent has its own potential advantages and disadvantages.12 Both agents are approved by the Food and Drug Administration (FDA) for this indication, but filgrastim is the most commonly used growth factor. Dosages are 10 mcg/kg/day (5–32 mcg/kg/day) for filgrastim and 250 mcg/m2/day for sargramostim. The combination of chemotherapy followed by a hematopoietic growth factor increases the number of PBSCs to a greater extent than either method alone. This approach is more expensive and is associated with more adverse effects than a growth factor alone, but the number of aphereses is reduced, and the additional chemotherapy may further reduce the tumor burden before transplant, which may reduce the likelihood of tumor cell contamination in the apheresis collection. Pegfilgrastim (pegylated filgrastim) has also been evaluated in the mobilization setting, either alone or after chemotherapy. Pegylation prolongs the half-life of filgrastim from 3 to 4 hours to 33 hours, allowing for single-dose administration. Both the 6- and 12-mg doses appear to be safe and at least as effective as filgrastim.13,14 Based on these studies, pegfilgrastim may be used in place of filgrastim for stem cell mobilization for patient convenience.
FIGURE 117-2 Schema for collection of peripheral blood progenitor cells after hematopoietic growth factor administration (top) or after chemotherapy and hematopoietic growth factor administration (bottom). Symbols with darker shading represent procedures performed only if adequate numbers of CD34+ cells have not been collected. (G-CSF, granulocyte colony-stimulating factor; GM-CSF, granulocyte-macrophage colony-stimulating factor.)
Plerixafor is a novel inhibitor of the CXCR4 chemokine receptor that is FDA approved as a mobilizing agent in combination with filgrastim. Its approval was based on two multicenter, randomized, double-blinded trials that compared filgrastim plus plerixafor or placebo as primary mobilization in patients with multiple myeloma or non-Hodgkin lymphoma.15,16 In both studies, patients received filgrastim 10 mcg/kg/day for 4 days; on the evening of the fourth day, they received either 240 mcg/kg of plerixafor or placebo. In both studies, a significantly larger proportion of the filgrastim plus plerixafor–treated patients were able to collect the target number of CD34+ cells in no more than two aphereses procedures compared with the filgrastim plus placebo group. These initial trials demonstrated that plerixafor is very effective in increasing the number of CD34+ cells available for primary mobilization of hematopoietic stem cells.
Because most patients are able to mobilize efficiently with filgrastim alone and plerixafor is expensive, transplant centers generally use some type of risk-adapted approach to identify which patients are appropriate candidates for plerixafor. One approach is to give plerixafor to patients with certain characteristics that have been associated with a high risk of poor mobilization.17,18 These characteristics include previous exposure to extensive chemotherapy, lenalidomide, or radiation, older age, hypocellular bone marrow, or low platelet counts. Another approach is to monitor CD34+ cell counts in the peripheral blood on day 4 or 5 of filgrastim administration because low numbers of CD34+ cells after filgrastim have been associated with mobilization failure. Patients who do not have a minimal number of CD34+ cells receive plerixafor.19–21 In general, these individualized approaches limit plerixafor administration to patients at high risk for poor mobilization.
In about 20% to 30% of autologous transplant candidates, an optimal number of CD34+ cells will not be obtained after the first attempt with standard mobilization regimens.22 Several strategies for overcoming the obstacle of poor mobilization have been evaluated, including remobilization with the same or higher doses of the same hematopoietic growth factor, a combination of hematopoietic growth factors (i.e., filgrastim and sargramostim), or a combination of chemotherapy and a hematopoietic growth factor. Bone marrow harvest is an option but often is of limited value. Plerixafor has been evaluated in patients who have failed primary mobilization. In a study of 115 patients who had failed at least one previous mobilization attempt, plerixafor and filgrastim were given with the objective of collecting at least 2 × 106 CD34+ cells/kg.23 Depending on diagnosis, about 60% to 75% of patients successfully mobilized with this regimen, which compares favorably with other secondary mobilization strategies. Although plerixafor with filgrastim may be effective for remobilization, it has been associated with a higher cost compared with other strategies, especially if multiple doses of plerixafor are required.24 The decision on which secondary mobilization regimen to be used should be based on patient-specific factors and clinician judgment.
Several studies show that the number of CD34+ cells infused correlates significantly with the rate of neutrophil and platelet recovery after high-dose chemotherapy.12 Rapid neutrophil recovery usually is observed in patients who receive at least 2 × 106 CD34+ cells/kg (body weight of recipient). More rapid platelet recovery is observed when at least 5 × 106 CD34+ cells/kg is transplanted compared with lower cell doses. As a result, most transplant centers use 2 × 106 CD34+ cells/kg as a minimum number to collect for autologous transplant, with an optimal target of 5 × 106 CD34+ cells/kg. For patients with multiple myeloma undergoing tandem transplants, cells for both transplants are collected before the first transplant. A minimum of 4 × 106 CD34+ cells/kg is required, and generally the entire cell dose collected is divided into two equal aliquots, one for each transplant.
Use of peripheral blood instead of bone marrow as a source of hematopoietic stem cells offers several clinical and economic advantages. The most clinically important advantage is that patients who receive mobilized PBSCs experience more rapid hematopoietic engraftment. Although engraftment of all lineages is more rapid when PBSCs are used, the most significant effect is observed with platelet recovery. Patients who receive mobilized PBSCs experience platelet recovery as much as 2 to 3 weeks earlier and require fewer platelet transfusions than those who receive bone marrow stem cells. As a result, patients usually are discharged earlier from the hospital, so the overall cost of autologous HSCT is reduced with the use of PBSCs. Another advantage is that the donor does not experience the discomfort associated with marrow aspirations and is not exposed to the risk associated with general anesthesia. PBSCs may be less likely to be contaminated with malignant cells compared with marrow stem cells. Finally, because PBSCs are collected from the mononuclear cell fraction, a fraction that also contains immunocompetent cells (e.g., natural killer [NK] cells and T lymphocytes), some investigators believe that infusion of PBSCs represents a form of “adoptive immunotherapy.” In this model, NK cells and lymphocytes targeted against tumor cells help to kill residual tumor cells. As a result of these clinical and economic advantages, peripheral blood has replaced bone marrow as the source of stem cells in the autologous setting.
Peripheral blood has also become the predominant source of hematopoietic stem cells in adult allogeneic HSCT.1,25 About 75% of allogeneic HSCTs performed in adults currently come from PBSCs harvested from normal donors. Concerns were raised initially over the safety and ethics of administering filgrastim to normal individuals volunteering as donors. Filgrastim is generally well tolerated. Short-term effects are similar to those seen in cancer patients receiving filgrastim (e.g., bone pain, headache, fever, arthralgias, malaise). Although there are concerns about increased risk of acute myelogenous leukemia (AML) in healthy subjects given filgrastim, no higher risk has been observed thus far.26 Because of the long latent period of drug-related AML and the very low incidence of AML in the general population, longer follow-up of thousands of healthy donors will be required to definitively conclude that an association between filgrastim and AML does not exist.
Randomized controlled trials and large registry studies show that patients who received allogeneic PBSC transplants from HLA-identical siblings experienced more rapid hematopoietic recovery and required fewer transfusions compared with patients receiving bone marrow.27 The difference in the rate of engraftment may be related to the threefold higher numbers of CD34+ cells infused in recipients of PBSC transplants. Although most of these studies did not report an increased risk of acute GVHD or transplant-related mortality in patients receiving allogeneic PBSC transplants, a higher risk of chronic GVHD has been observed. In a meta-analysis of nine randomized trials evaluating HLA-matched sibling donor transplants in adult patients, the risk of chronic GVHD was nearly twofold higher for patients who received allogeneic PBSC transplants compared with those who received bone marrow transplantation (BMT). However, the risk of relapse was higher in the patients receiving BMT, and no significant difference in overall survival was observed in the meta-analysis, although in some individual studies, disease-free and overall survival were improved with PBSC. When patients were analyzed based on disease status at the time of transplant, patients with hematologic malignancies at high risk of relapse had a better overall survival when transplanted with PBSC as compared with those who received BMT, which may be related in part to the lower risk of relapse in patients who received PBSC transplants.27 The Blood and Marrow Transplant Clinical Trials Network recently reported the results of a trial that randomized 551 patients to allogeneic PBSC or bone marrow from matched unrelated donors.28 At 2 years after transplant, there were no differences in overall survival, relapse, or mortality not related to relapse. Neutrophil and platelet engraftment were more rapid, and the risk of graft failure was reduced in the PBSC transplants compared with patients receiving BMT. The risk of acute GVHD was similar, but a higher incidence of chronic GVHD was reported in patients who received PBSC transplants. Two-year survival is an early outcome, and further follow-up will need to be done to determine if these results are maintained over time. Selection of the optimal source of hematopoietic stem cells for an individual patient should be based on the risk of relapse, chronic GVHD, graft failure, and donor preference. It is also important to note that most patients treated in the randomized trials of bone marrow versus peripheral blood transplants received myeloablative conditioning (MAC) regimens (discussed later), and it is unknown whether these results can be extrapolated to RIC regimens.
Several small studies have reported the engraftment and outcomes of patients receiving allogeneic bone marrow from donors who received filgrastim for 3 to 4 days before harvest. The use of the filgrastim was hypothesized to increase the yield of bone marrow from healthy donors. A meta-analysis of studies comparing filgrastim stimulated bone marrow to PBSCs showed similar rates of engraftment, acute GVHD, relapse, and overall survival but a greater risk of chronic GVHD with PBSCs. These initial studies suggest that filgrastim-stimulated bone marrow may result in early engraftment similar to PBSCs without the higher risk of GVHD.29 A large prospective randomized trial is comparing filgrastim–mobilized PBSCs with filgrastim-stimulated bone marrow.
In addition to bone marrow and peripheral blood, hematopoietic stem cells are found in UCB. UCB is an attractive source for several reasons.30 Because the stem cells are collected from placental blood, there is no risk to the mother or the baby and a very low risk of transmissible infectious diseases, such as cytomegalovirus and Epstein-Barr virus. The cells are available immediately because the donor does not have to be located and the stem cells harvested. UCB initially was obtained from siblings, but now recipients of transplants from unrelated donors account for almost all patients who receive UCB transplants. More than 600,000 UCB grafts are available in more than 100 UCB banks, and more than 20,000 unrelated UCB transplants have been performed worldwide.30
Recipients of UCB transplants usually receive a CD34+ cell dose more than 1 log lower than that given to recipients of BMT, and this difference in CD34+ cell dose may explain the delayed engraftment in recipients of UCB transplants. The number of infused total nucleated and CD34+ cells correlates with outcomes after UCB transplantation. Although no randomized comparisons have been performed, many retrospective studies have compared outcomes of UBC transplantation with more traditional stem cell sources such as bone marrow or PBSCs. Analysis of data from the CIBMTR and the New York Blood Center showed similar survival in children with acute leukemia who underwent either unrelated HLA-mismatched UCB transplantation or unrelated HLA-matched BMT. Children who received HLA-matched UCB transplants had better outcomes than those who received HLA-matched BMTs. However, higher transplant-related mortality was observed in children transplanted with a low UCB cell dose and a mismatched UCB graft.31 Similar studies of adults with hematologic malignancies have been conducted. The CIBMTR compared outcomes for adults with acute leukemia who were transplanted with unrelated BM or PBSC versus UCB. Both overall survival and leukemia-free survival were similar in all transplant groups. The risk of both acute and chronic GVHD was lower in UCB recipients compared with PBSC, and the risk of chronic GVHD was lower in UCB compared with bone marrow. However, transplant-related mortality was higher after UCB as compared with other stem cell sources. These data support the use of UBCs as a source of stem cells when matched PBSCs or bone marrow are not immediately available.32
A major limitation of UCB transplants is the small volume of blood collected, usually 60 to 150 mL with resultant low numbers of CD34+ cells. Although the relatively low numbers of hematopoietic cells may be adequate for hematopoietic engraftment in children and small adults, it may not be adequate for larger recipients. Efforts to expand the number of hematopoietic stem cells include “pooling” 2 or more units of UCB for one recipient (referred to as double cord transplant). The Seattle and Minnesota groups recently published their experience in more than 500 patients older than 10 years of age who received a matched related donor, matched unrelated donor, mismatched unrelated donor, or double cord transplant. Leukemia-free survival was similar in all groups, but the double cord transplant recipients had a higher risk of transplant-related mortality. Although the role of double cord transplantation has not yet been fully defined, the results of this study suggest that pooled UBCs may provide an option for patients in which no other appropriate donors are available.33
APPROACHES TO ERADICATE MALIGNANT CELLS
Conditioning Regimens
The purpose of the pretransplant conditioning regimen (also called the preparative regimen) depends on the type of transplant and the indication for its use. In the autologous setting, pretransplant conditioning is used to eradicate malignant cells. This is also the case in allogeneic transplantation for malignant diseases, but the conditioning regimen also serves a dual purpose to suppress the recipient’s immune system to allow for donor cell engraftment. Two types of conditioning regimens are used, myeloablative and reduced intensity. Myeloablative conditioning (MAC) regimens contain very high doses of chemotherapy with or without radiation that would lead to life-threatening or fatal myelosuppression if hematopoietic stem cells were not infused. Patients undergoing autologous transplantation receive MAC regimens. Reduced-intensity conditioning (RIC) regimens consist of lower doses or different types of chemotherapy or lower doses of radiation than used in MAC regimens. RIC regimens were developed after the observation was made that some of the antitumor effect of the allogeneic transplant was mediated by a reaction between the donor’s immune system and the recipient’s cancer cells. This meant that very high doses of chemotherapy, radiation, or both may not be needed. Because RIC regimens use lower doses of chemotherapy or radiation or less toxic drugs, older patients and those with comorbidities are now able to undergo allogeneic transplant. Both types of regimens are discussed in detail below.
Myeloablative Regimens
MAC regimens usually include at least one anticancer drug with a relatively steep dose-response curve and myelosuppression as their dose-limiting toxicity, such as alkylating agents. Cyclophosphamide, melphalan, busulfan, and carmustine are examples of chemotherapy agents commonly used in MAC regimens. Other agents are usually added that have additive or synergistic effects with these alkylating agents in specific types of cancers; other alkylating agents have also been used. Table 117-1 lists chemotherapeutic agents that are frequently used in MAC regimens as well as the doses used and their dose-limiting toxicity in the transplant setting.
TABLE 117-1 Dose-Limiting Nonhematologic Toxicities for Selected Chemotherapeutic Agents Included in Myeloablative Conditioning Regimens in Hematopoietic Stem Cell Transplantation
Total-body irradiation (TBI) is also used in some pretransplant conditioning regimens. In patients with malignant disease, the rationale of TBI is to eradicate malignant cells located in areas inaccessible to the systemic circulation and thus to the chemotherapeutic agents. TBI also has significant immunosuppressive activity. TBI doses for MAC regimens range from 10 to 15 Gy (1,000–1500 rads), which is more than twice the lethal dose of radiation for a normal person. TBI in these doses is typically fractionated (split over several days, once or twice a day) rather than given as a single-dose. Fractionated TBI has an improved therapeutic ratio compared with single-dose administration, that is, destruction of more leukemic cells and marrow stem cells while sparing other normal tissues. The acute toxicities of TBI consist of fever, nausea, vomiting, diarrhea, mucositis, and tender swelling of the parotid gland. Long-term complications of TBI-containing regimens include cataract formation, growth retardation, carcinogenesis, permanent reproductive sterility, and secondary malignancies.
Two of the most commonly used MAC regimens for allogeneic transplant are cyclophosphamide and TBI (CyTBI) or busulfan and cyclophosphamide (BuCy). When given with TBI, cyclophosphamide is usually given first as two doses of 60 mg/kg/day followed by TBI. In the original BuCy regimen, busulfan was given orally at a dosage of 1 mg/kg orally every 6 hours for 16 doses on days –9 to –6 (4 mg/kg/day for 4 days; day 0 being the day of transplant) followed by four doses of cyclophosphamide given IV once daily at a dosage of 50 mg/kg on days –5 to –2. In one widely used modification of the regimen (BuCy2), the total cyclophosphamide dosage is reduced from 200 (50 × 4) to 120 (60 × 2) mg/kg. Plasma busulfan concentrations are monitored at some centers because studies suggest that systemic exposure correlates with outcome, and use of a targeted busulfan and cyclophosphamide preparative regimen may improve patient outcome.34 The IV form of busulfan (Busulfex®) reduces some of the interpatient variability in systemic exposure and may also reduce the risk of hepatotoxicity. The dose of IV busulfan approved for pretransplant conditioning regimens is 0.8 mg/kg every 6 hours for 4 days, although once-daily dosing regimens have also been developed.
Several prospective randomized studies have compared CyTBI with BuCy in patients with acute or chronic myeloid leukemia (CML) undergoing allogeneic matched related donor HSCT.35,36 Early results of these studies showed that BuCy had similar or greater antileukemic activity than CyTBI in patients with CML and that CyTBI was associated with slightly better disease-free survival in patients with AML. However, with longer follow-up, similar survival rates were observed. In a large retrospective analysis of the CIBMTR in patients with myeloid malignancies receiving unrelated donor transplants, overall and disease-free survival were not significantly different between the two regimens.37 Long-term toxicities between the two regimens appear to be comparable. Since the publication of these studies, several improvements in clinical practice have been made (e.g., better supportive care, more specific HLA typing methodologies, use of PBSCs) that may have some effect on the comparison of these two commonly used regimens. For example, use of the IV form and pharmacokinetic monitoring of busulfan may optimize its use, giving an advantage to the use of BuCy. In addition, other MAC regimens have been developed that may be safer or more effective. Without definitive data showing the superiority of one regimen over another, the choice of regimens before allogeneic transplant generally is based on the experience of the transplant center, patient characteristics, diagnosis, and disease status.
Conditioning regimens used in autologous HSCT are exclusively myeloablative and generally include at least one alkylating agent with other agents added that may have specific activity against the tumor type being treated. TBI usually is not included in the conditioning regimen in patients who have received prior radiotherapy. MAC regimens used in patients with lymphoma generally include different combinations of cyclophosphamide, carmustine, etoposide, and cytarabine. Rituximab is commonly added to the high-dose chemotherapy regimen in patients with CD20-positive lymphomas. Many transplant treatment regimens also use rituximab at the time of stem cell collection to reduce the number of CD20-positive lymphoma cells in the stem cell product being collected. The availability of anti-CD20–radiolabeled monoclonal antibodies, iodine-131 tositumomab and yttrium-90 ibritumomab, offers the potential to deliver targeted radiation to CD20-positive tumor cells and less to normal organs. Based on the results of several promising phase II studies with these two agents, the Blood and Marrow Transplant Clinical Trials Network conducted a prospective comparative trial randomizing patients with diffuse large B cell lymphoma to receive high-dose chemotherapy with rituximab or iodine-131 tositumomab followed by autologous HSCT.38 Progression-free survival (PFS) and overall survival were not significantly different between the two groups, and thus these agents are not used routinely as part of conditioning for patients with lymphoma undergoing autologous HSCT. Single-agent melphalan (200 mg/m2) is the standard conditioning regimen for patients undergoing autologous HSCT for myeloma. Studies are ongoing evaluating the addition of newer agents (e.g., bortezomib).
Reduced-Intensity Conditioning Regimens
Donor T cells contribute to the tumor cell kill and prevention of relapse observed after allogeneic HSCT, an effect referred to as the graft-versus-malignancy (GVM) effect. Evidence for the GVM effect is based on retrospective studies showing that patients who developed GVHD had a lower risk of leukemic relapse than those who did not develop GVHD. However, the overall survival rate was not different because of the increased nonrelapse mortality associated with GVHD. Other anecdotal evidence supporting a T cell–mediated GVM effect was the increased risk of relapse found with T cell–depleted transplants compared with unmodified transplants and the efficacy of donor lymphocyte infusions (DLIs) in producing responses in patients who have relapsed after allogeneic HSCT.
RIC regimens containing lower doses of chemotherapy or radiation or less toxic agents were developed to take advantage of the GVM effect but with a lower incidence of regimen-related toxicity than that of MAC regimens. Animal data demonstrated that MAC was not required for engraftment of donor cells (the other important role of conditioning in allogeneic HSCT), thus paving the way for the evaluation of RIC in humans.39,40 The major advantage of RIC is that potentially curative transplants can be offered to patients who typically would not be considered for allogeneic HSCT because of their unacceptably high risk of transplant-related complications because of increased age or moderately compromised organ function. Use of RIC regimens has steadily increased in patients aged 50 and older.1 In addition, because of the lower rate of toxicity, allogeneic HSCT with RIC can be offered to patients who have relapsed after traditional myeloablative autologous or allogeneic transplants. About 30% of allogeneic transplants are now being performed with RIC regimens.1
Because RIC regimens may not be completely myeloablative, host hematopoiesis can persist and lead to mixed chimerism (blood cells from both donor and recipient are present) (Fig. 117-3).39 Several studies have reported significant correlations between donor T-cell chimerism levels and the risk of graft rejection, GVHD, and relapse. For example, a low percentage of donor T and NK cells present on day 14 has been associated with graft rejection, but high T-cell donor chimerism on day 28 has been associated with acute GVHD. Achievement of full donor chimerism was associated with better PFS. These data suggest that monitoring donor chimerism after transplant may allow early interventions to prevent graft rejection or relapse.
FIGURE 117-3 Schema for nonmyeloablative transplantation for hematologic malignancy. Recipients (R) receive a reduced-intensity conditioning regimen and an allogeneic hematopoietic stem cell transplant (HSCT). Initially, mixed chimerism is present with the coexistence of donor (D) cells and recipient-derived normal and leukemia/lymphoma (RL) cells. Donor-derived T cells mediate a graft-versus-host hematopoietic effect that eradicates residual recipient-derived normal and malignant hematopoietic cells. Donor lymphocyte infusions (DLIs) can be administered to enhance graft-versus-malignancy effects.
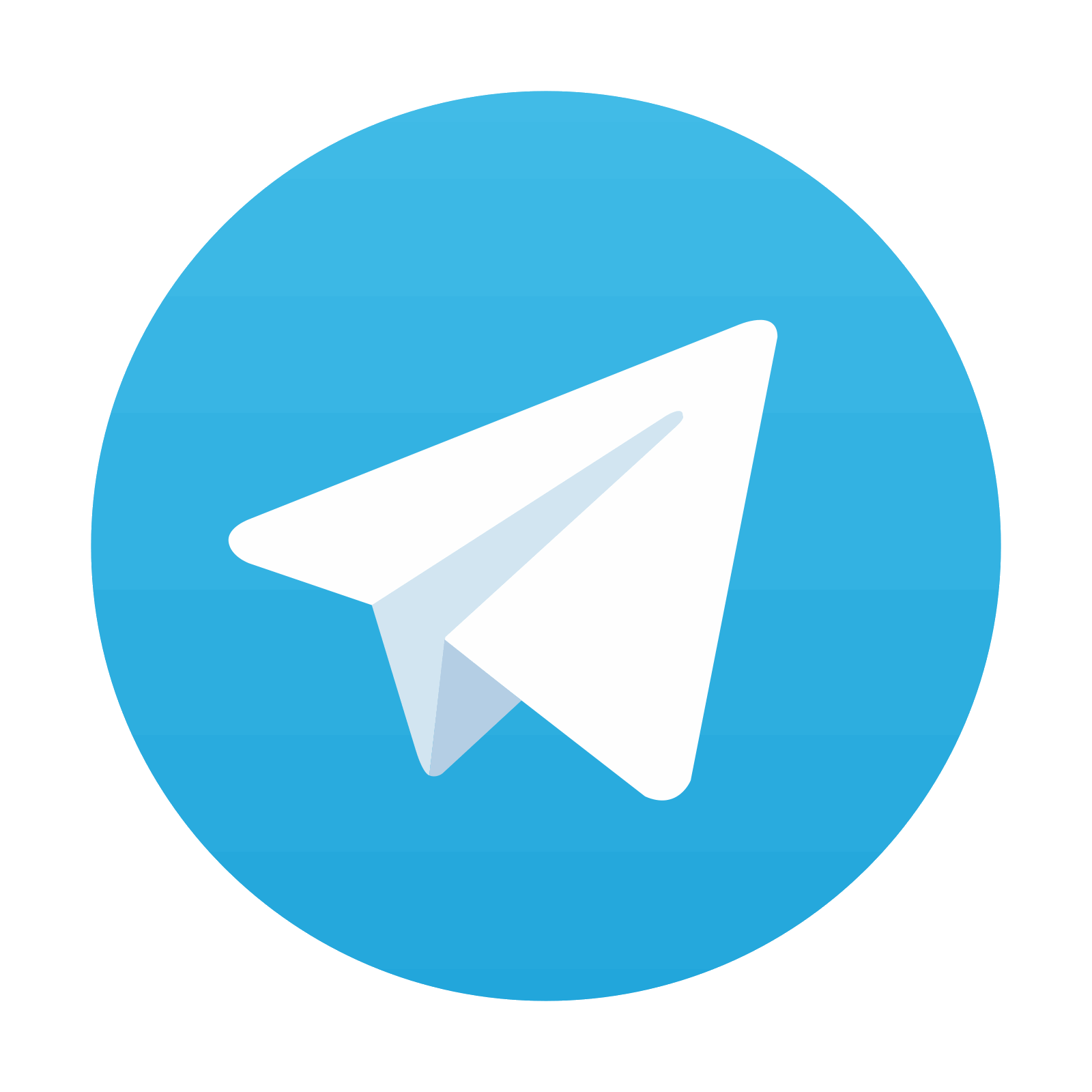