Groundwater Purification
Nicholas P. Cheremisinoff
Chris Brown
Water purification is the process by which undesired chemical compounds, organic and inorganic materials, as well as biological contaminants are removed from water. There are multiple processes that serve this purpose. Selection and costs depend on the type and nature of contaminants, the extent of contamination, the cleanup or purification levels that are defined or established from statutory standards, and the intended use of the purified water. A major purpose of water purification is to provide potable drinking water. Water purification is also intended to meet the needs of medical, pharmacological, chemical, and industrial applications for clean and potable water as well as agricultural use. Purification reduces the concentration of contaminants such as suspended particles, parasites, bacteria, algae, viruses, fungi, as well as chemical constituents introduced from manufacturing operations that are often the result of spills and other unintentional releases to ground and surface water sources.
Water purification operations are implemented on scales spanning from the large (eg, for an entire city) to the small (eg, for individual households). Most communities rely on natural bodies of water as intake sources for water purification and for day-to-day use. In general, these resources can be classified as groundwater or surface water and include underground aquifers, creeks, streams, rivers, and lakes. Oceans and saltwater seas have also been used as alternative water sources for drinking and domestic use. This chapter provides an overview of water purification technologies that are commonly applied in industrial settings and remedial activities. Available technologies must be carefully assessed in terms of their suitability for an intended application. Although the processes can be classified generically, many purification applications require unique engineering solutions to meet water quality targets.1

When groundwater has been adversely impacted, a variety of sciences, strategies, technologies, and actions are needed to assess human and ecological risks from contamination. The first step in assessing impacts requires an environmental site assessment. The goal of an environmental site assessment is to identify recognized environmental conditions, referring to the presence of any hazardous or petroleum substances on a property under conditions that indicate release into the ground, groundwater, or surface water.2
In the United States, as an example, the control and prevention of the entry of hazardous substances into the environment is the objective of several acts of congress. Rules regulating various aspects of hazardous waste can be attributed to the Toxic Substances Control Act; Clean Water Act; Clean Air Act; Federal Insecticide, Fungicide, and Rodenticide Act; Safe Drinking Water Act; Resource Conservation and Recovery Act (RCRA); and the Comprehensive Environmental Response, Compensation, and Liability Act (CERCLA). The RCRA and CERCLA are the two that are most often associated with environmental site assessments. Many, if not most, state agencies publish risk-based cleanup criteria for industrial sites and recognize “mixing zone” concepts that allow stable contaminated plumes to attenuate in place so long as surface water and drinking water resources are protected. The ASTM International has also developed a risk-based corrective action standard for chlorinated solvents that is similar to the standard developed for fuel.3
The nature and extent of a site’s groundwater contamination must be defined in part with a conceptual model. The investigator needs to develop a useful conceptual
site model or update an existing one and determine what human or ecological receptors may be at risk and how to limit their exposure to the contamination. An accurate conceptual site model is critical to evaluating the true risk of contamination as well as the possibilities and limitations of site remediation strategies. A complete model should include a visual representation of contaminant source and release information, site geology and hydrology, contaminant distribution, fate and transport parameters, and risk assessment features such as current and future land use and potential exposure pathways and receptors. The conceptual site model should be developed as a part of the site investigation or feasibility study phase of site remediation. Many interim remedial systems have been installed and are operating without a well-defined model, oftentimes leading to major cost overruns or inability to achieve cleanup goals within reasonable time periods. Some remedial systems were designed based on an initial model that requires updating based on recent operations and monitoring data. Changes in land use, or changes in the enforcement of institutional controls, can also alter the exposure and risk assumptions of the model. It is important to recognize that the conceptual site model is intended to be a dynamic representation of site conditions based on a continual influx of information from the site. The following are important elements of a conceptual site model.
site model or update an existing one and determine what human or ecological receptors may be at risk and how to limit their exposure to the contamination. An accurate conceptual site model is critical to evaluating the true risk of contamination as well as the possibilities and limitations of site remediation strategies. A complete model should include a visual representation of contaminant source and release information, site geology and hydrology, contaminant distribution, fate and transport parameters, and risk assessment features such as current and future land use and potential exposure pathways and receptors. The conceptual site model should be developed as a part of the site investigation or feasibility study phase of site remediation. Many interim remedial systems have been installed and are operating without a well-defined model, oftentimes leading to major cost overruns or inability to achieve cleanup goals within reasonable time periods. Some remedial systems were designed based on an initial model that requires updating based on recent operations and monitoring data. Changes in land use, or changes in the enforcement of institutional controls, can also alter the exposure and risk assumptions of the model. It is important to recognize that the conceptual site model is intended to be a dynamic representation of site conditions based on a continual influx of information from the site. The following are important elements of a conceptual site model.
Source and Release Information
The model should include a description of the source of contamination and what is known about the timing and quantity of the release. Most site characterizations begin by locating areas where chemical contaminants were originally released to the subsurface. In many cases, the distinct source of contamination is known to be a former underground storage tank, disposal pit, a leaking pipeline, a spill, etc; however, many industrial source areas are dispersed and sometimes difficult to delineate. For example, oil/water separators and sanitary and storm sewers have historically received chlorinated solvents from process operations or various plant maintenance shops. At such sites, it may be impossible to pinpoint the exact source of contamination.
The timing and amount of chemical contaminants released are equally difficult to estimate. Historical records on chemical uses are sometimes difficult to obtain, and if they exist are generally found in Phase I Installation Restoration Program documents developed in the United States in the early 1980s. Many sites as examples used chlorinated solvents like trichloroethylene (TCE), which was used for decades at some operations before it was phased out in the early 1980s. Such chemicals may not have been widely used at a facility for nearly 20 to 30 years. This fact is important when evaluating the fate and transport of chlorinated solvents or any chemical contaminant and is especially important when estimating degradation rates based on the breakdown products of certain chemicals like the chlorinated solvents.
Geological and Hydrogeological Characterization
The model must include a complete description of the site geology and hydrogeology. The descriptions should include the following at a minimum:
A general description of site geology including major soil strata that are impacted by or influence the migration of contaminants. Strata thickness, lateral extent, continuity and depositional features should be described.
Physical and chemical properties of subsurface materials such as sieve analysis, bulk density, porosity, and total organic carbon
Geological or man-made features, which may provide preferential migration of chemicals, dense nonaqueous phase liquids (DNAPLs), solvent vapors, or dissolved contaminants
Depth to groundwater, seasonal variations, recharge, and discharge information including interactions with surface waters
Ranges of hydraulic gradients (horizontal and vertical)
Ranges of hydraulic properties (eg, hydraulic conductivity, storage coefficient, effective porosity, seepage velocity)
Geochemical properties influencing the natural biodegradation of the chemical contaminants
This may need to be updated to reflect current estimates of these properties based on site remediation experience. For example, the hydraulic properties of an aquifer can be more accurately estimated after a groundwater extraction system has operated several months. On sites where natural attenuation has been selected as the groundwater remedy, tracking the movement (or stability) of the contaminant plume provides essential information that can be introduced into an updated model.
Contaminant Distribution, Transport, and Fate
The model should include a summary of the chemical, physical, and biodegradation properties of key contaminants of concern and describe their distribution, movement, and fate in the subsurface environment. Descriptions should include the following:
Chemical and physical properties of the chemical contaminants that impact subsurface transport (eg, partitioning coefficients, solubility, vapor pressure, Henry’s law, density, viscosity)
Estimates of the phase distribution of each contaminant in the saturated and unsaturated zone
Temporal trends in contaminant concentrations in each phase
Geochemical evidence of contaminant natural attenuation processes (destructive and nondestructive)
Geochemistry Impacting Natural Biodegradation
Under certain conditions, geochemical parameters may favor natural biodegradation of some chemicals. Examples of these can be found with chlorinated solvents. Geochemical indicators such as dissolved oxygen, nitrate, iron, manganese, sulfate, methane, and hydrogen ion concentrations should be reported in the conceptual site model. In the case of groundwater contamination caused by chlorinated solvents, the relative distribution of primary solvents such as tetrachloroethene (PCE) and TCE and daughter products such as 1,2-dichloroethane (DCE) and vinyl chloride should be documented in relation to the geochemical profile of the site.
Risk-Based Cleanup Goals and Screening Level Evaluations
Important considerations for defining risk-based goals are the following:
Determining the risk-based screening levels that are appropriate for a contaminated site
Developing site-specific cleanup goals based on realistic exposure scenarios at the site
Estimating the average exposure concentration as opposed to the maximum concentration at the site
Once a conceptual site model has been devised, defining the source of chemical contamination, potential pathways, and potential receptors, the task of defining risk-based cleanup objectives may begin. This can be approached as a two-step process involving the following actions:
First, an initial comparison of potential exposure concentrations to conservative industrial screening levels for each contaminant of concern can be made. For sites with potential discharges to surface water bodies, a comparison to ecological screening levels may be deemed appropriate.
Second, any contaminant exceeding conservative screening levels can be evaluated using more realistic, site-specific exposure assumptions to determine if an unacceptable human health or ecological risk could or potentially exist.
A two-step approach provides flexibility to replace potentially conservative, nonsite-specific exposure assumptions with site-specific information while still providing the same level of human health and environmental resource protection. The investigator is likely to encounter increasingly complex levels of data collection and risk along the process. The evaluation will need to be performed in order to establish the type and magnitude of remediation required to reduce or eliminate unacceptable risks at a particular site. This may be accomplished by replacing nonsite-specific (ie, default) assumptions about how chemicals behave in the environment and how receptors may be exposed, with site-specific data and assumptions that are more representative of actual site conditions and realistic exposure pathways for human and ecological receptors.
A screening level evaluation provides a means of identifying whether a particular chemical warrants additional risk evaluation. Screening levels are conservative (health protective), generic cleanup criteria that define the residual amount of a contaminant that can remain onsite and not present an unacceptable risk to potential receptors. For sites with the potential for discharge to surface waters, ecological screening levels are appropriate. Industrial screening levels for human receptors are generally based on reasonable maximum exposure assumptions and can be either health protective or designed to mitigate nuisances associated with chemical contamination (eg, taste and odor). In order to select (or develop) appropriate screening levels, information about the current and potential future land and groundwater uses at/or down-gradient from the affected site must be thoroughly documented in the conceptual site model. It is common practice to consider screening levels for industrial land use scenarios over prolonged periods of say 25 years and by taking into consideration exposure to all contaminated media. Many published industrial screening levels assume ingestion of onsite groundwater by a specific receptor group (eg, industrial onsite workers). Such assumptions may or may not be realistic; however, such conservative screening levels may be appropriate if groundwater use cannot be absolutely controlled through pumping restrictions or pump and treat strategies.
Once the appropriate land use category has been defined, the types of exposure pathways to be considered in the screening evaluation should be defined. An essential step in defining appropriate screening levels is determining the risk target level. Acceptable target risk ranges for carcinogens (eg, chlorinated solvent contamination and in particular vinyl chloride) fall between 10-6 and 10-4.1 The risk ranges are equivalent to an added lifetime cancer risk of 1 in 1 000 000 to 1 in 10 000 for people exposed to site contamination. Screening levels for carcinogens typically are based on an extremely protective 10-6 target risk level that is referred to as a de minimis risk level, meaning that a 1 in 1 000 000 risk level is so small as to be of negligible concern. A 10-6 target risk level should be considered health protective, given that the “normal background level” of cancer in the general population is about 1 in 3 persons (30%-35%). The US Environmental Protection Agency (EPA) reports that for
carcinogens, a 10-6 target risk level for individual chemicals and pathways generally will lead to cumulative risks within the 10-4 to 10-6 risk range for the combinations of chemicals typically found at contaminated sites.4 In addition to potential human receptors, a screening level evaluation should consider potential ecological receptors and other environmental resources that could be impacted by site contaminants.5
carcinogens, a 10-6 target risk level for individual chemicals and pathways generally will lead to cumulative risks within the 10-4 to 10-6 risk range for the combinations of chemicals typically found at contaminated sites.4 In addition to potential human receptors, a screening level evaluation should consider potential ecological receptors and other environmental resources that could be impacted by site contaminants.5
Once applicable screening levels are identified, the evaluation process consists of comparing representative exposure-point concentrations from recent site sampling events to applicable screening levels. It is important to use the most recent site contamination data. It is good practice to evaluate the two most recent sampling events and a comparison of maximum detected site concentrations to applicable screening levels. The use of statistically averaged site concentrations may be appropriate at some sites.

The DNAPLs are liquids that are only slightly soluble in water and therefore exist in the subsurface as a separate fluid phase immiscible with both water and air. Common types include wood treating oils such as creosote, transformer and insulating oils containing polychlorinated biphenyls, coal tar, and chlorinated solvents such as TCE and PCE with other, less frequently encountered being mercury and certain crude oils. Most DNAPL products are toxic and some compounds are highly mobile in the subsurface and groundwater. Unlike light nonaqueous phase liquids such as gasoline and heating oil (products that are less dense than water), DNAPLs are denser than water and have the ability to migrate to significant depths below the water table. There they slowly dissolve into flowing groundwater, giving rise to aqueous phase plumes. Releases of DNAPLs at the ground surface can result in longterm contamination of both the unsaturated and saturated zones at a site. Although DNAPLs have been produced and heavily used in industry since the beginning of the 20th century, their importance as soil and groundwater contaminants was not recognized or understood until the 1980s. This lack of recognition was partly due to the fact that the analytical methods and tools required to detect low concentrations of organic compounds in groundwater were not widely available or used until relatively recently.
![]() FIGURE 57.1 Conceptual illustration of a migration of dense nonaqueous phase liquid (DNAPL) released to ground surface. Abbreviation: GW, ground water. |
Essentially, all DNAPLs can be characterized by their density, viscosity, interfacial tension with water, component composition, solubility in water, vapor pressure, and wettability. When a DNAPL is released at the ground surface, it will migrate both vertically and laterally in the subsurface (Figure 57.1). The amount of residual DNAPL retained by a typical porous medium such as silt, sand, and gravel may range between 5% and 20% of the available pore space.
Remedial strategies and technologies are selected by taking into consideration the effectiveness, practicability, durability, and likely costs and benefits of the various remediation options. The EPA seeks to ensure that within this framework, the most sustainable approach to remediation is selected. The area of contamination that is the focus of remediation may vary from site to site but typically involves one or both of the following:
A DNAPL present within the source zone along with the associated aqueous and sorbed phase contamination in the source zone. If unsaturated media is involved, vapor phase contamination may also be addressed.
Aqueous phase contamination present downstream of the DNAPL source zone. This will typically have sorbed phase contamination (on the soil or aquifer materials) associated with it and may include vapor phase contamination in unsaturated media.

The selection of effective remediation strategies is often not straightforward due to the nature of chlorinated compound contamination. Site soil conditions can limit the selection of a treatment process. Process-limiting characteristics such as pH or moisture content may sometimes be adjusted. In other cases, a treatment technology may be eliminated based on the soil classification (eg, particlesize distribution) or other soil characteristics. Soils are inherently variable in their physical and chemical characteristics. Usually, the variability is much greater vertically than horizontally, resulting from the variability in the processes that originally formed the soils. The soil variability, in turn, will result in variability in the distribution of water and contaminants and in the ease with which they can be transported within, and removed from, the soil at a particular site. The following are common treatment technologies.
Soil Excavation, Treatment, and Disposal
If a discrete source zone containing DNAPL is identified and isolated, then a possible remediation strategy is the excavation of contaminated soil. Soil excavation methods are well established; after soil containing DNAPL has been removed from a source zone, it may be properly disposed, or treated—strategies include disposal in hazardous waste landfills, biodegradation cells, thermal desorption, chemical oxidation. Soils contaminated with chlorinated solvents generally cannot be disposed of in landfills unless they can be shown to be nontoxic based on the Toxic Characteristic Leaching Procedure.
Successful contaminant source removal/excavation starts with the collection of a sufficient number of soil/sediment samples to adequately characterize the extent of the contaminant source area, including variations in lithologies. A variety of equipment has been used to excavate contaminated soil or remove contaminated media. Typical machinery includes backhoes and track hoes for soil excavations and storage tank removals. Small track hoes are used inside buildings to excavate contaminated soils inside a building. Vacuum trucks are used to excavate contaminated soil, remove contaminated sediments from soakage pits and cleanout contaminated sludge and wastewater from septic tanks. Vacuum trucks can be used for shallow excavations under building floor slabs where access is limited. At some sites where remediation is performed indoors, a portion of the floor slab is cut out at contaminant source areas and the vacuum truck is parked near the facility, where the vacuum hose can access the excavation via the service door. Contaminated soil is removed down to the water table and then the excavation is backfilled with grout or sand and the floor slab restored.
Soil Vapor Extraction
Soil vapor extraction (SVE) strategies were developed to remove volatile contaminants from unsaturated soils. The SVE is a frequently used remedial strategy in situations where excavation is not feasible due to the presence of physical obstructions (eg, buildings, utilities, trees, etc), or where the extent of soil contamination is extensive. The SVE systems can be very effective at minimizing indoor vapor intrusion by keeping the contaminated soil vapors closer to the source area. The technology involves the application of a vacuum to slotted pipes in the vadose zone to draw air through contaminated material. The air flow volatilizes contaminants from the DNAPL, soil, and aqueous phases. The goal of most SVE operations at DNAPL sites is to remove sufficient contaminant mass so that water percolating through the vadose zone will no longer dissolve contaminants and transport them to the water table at concentrations exceeding regulatory limits. An SVE is capable of achieving the goal in relatively homogeneous, coarse-grained soils where air can rapidly move through the contaminant zone. An SVE is not as effective in mobilizing contaminants from the capillary fringe or in fine-grained or very moist strata. Slow diffusion of contaminants from residual DNAPL entrapped in these zones limit restoration rates.
Enhanced Methods of Soil Vapor Extraction
Multiphase extraction refers to remediation strategies that incorporate combinations of groundwater pumping, DNAPL removal, and SVE using a single well to extract both liquid and vapor. Depending on the site, the depth at which SVE can be applied is increased by lowering the water table with groundwater extraction. At sites with mobile DNAPL, a low rate of groundwater pumping can be applied to lower the water table while applying SVE to enhance liquid migration toward the well and to remove volatiles from the expanded unsaturated zone. The multiphase extraction strategy is applicable at sites with permeable soils, where the majority of the DNAPL is trapped within the upper 10 ft (3.05 m) of the aquifer. Multiphase
extraction is less successful at sites with low-permeability soils because dewatering at the well may not result in a significant reduction in the water saturation level of the soil. In this case, the air permeability of the soil will not be improved by application of simple dewatering.
extraction is less successful at sites with low-permeability soils because dewatering at the well may not result in a significant reduction in the water saturation level of the soil. In this case, the air permeability of the soil will not be improved by application of simple dewatering.
Another strategy is applying thermal enhancements to SVE system. In situ heating methods that have received attention are aimed at reducing soil moisture and thereby enhancing SVE effectiveness by extending SVE influence into shallow aquifers. In situ heating strategies offer potential to increase the permeability of silt and clay soils and increase the removal efficiency of SVE. Steam injection combined with vacuum extraction may also be considered an approach to remove volatile DNAPL from the vadose and shallow groundwater zones. When high pressure “dry” steam is injected into contaminated soil, volatile chemicals with boiling points lower than that of water are vaporized. The SVE wells are then used to remove the resulting vapor. This process has been applied at smaller sites with relatively uniform permeable soils but may not be as effective in layered soils. Other methods use resistive or radio frequency heating for increasing soil temperatures and volatilizing DNAPL residuals. The most promising of the resistive heating technologies is a sixphase system, which uses metal and graphite electrodes to set up an alternating electrical current in the soil.6 This strategy is limited to sites with soil moisture levels above 10% because water is required to conduct the electricity and to create resistive heating. Resistive heating can reach a maximum temperature of 100°C, which boils away the groundwater and can volatilize residual DNAPLs. Once volatilized, volatile organic compounds are removed by a concurrently operating SVE system. Radio frequency heating is a process that uses electrodes placed in the subsurface to deliver radio frequency energy that excite molecular motion and induce heating (in the same way that microwave ovens heat food). Radio frequency heating has the potential to heat soil to temperatures in excess of 200°C and can be used to volatilize higher boiling point compounds such as mixtures of jet fuel and solvents. The SVE wells are then used to remove the resulting vapor.7
In situ Air Sparging
In situ air sparging is the process of injecting air into the saturated zone with the objective of stripping the contaminants from the dissolved phase and transferring these compounds to a vapor phase. The strategy competes with conventional baseline technologies of pump-and-treat and pump-and-treat combined with SVE. Vertical well air sparging and in well recirculation technologies have been implemented at a number of sites.
An air sparge network consists of sparge points designed to deliver air to a specific zone of contaminated groundwater. Air compressors deliver contaminant-free air under pressure to the target zones. The vapor migrates upward from the saturated zone to the unsaturated zone. The vapor phase is then vented through the unsaturated zone to the atmosphere and typically uses an SVE in the unsaturated zone to more effectively control, treat, and remove the vapor plume from the unsaturated zone.
Volatile, semivolatile, and nonvolatile organic contaminants in dissolved, free-phase, sorbed, and vapor phases can be treated using air sparging. Air sparging is applicable for the treatment of less volatile and/or tightly sorbed chemicals that could not be remediated using vapor extraction alone. Contaminants affected by the volatilization and biodegradation processes of air sparging include various fuels (eg, gasoline, diesel, jet fuel), oils and greases, and the chlorinated solvents (PCE, TCE, DCE, etc). Some commercially available systems (eg, BioSparge®) employs an ozone generator with the air sparging technique to extend the capabilities of the technology to chlorinated phenols (pentachlorophenol [PCP]), alcohols, ketones, and other industrial solvents. The injected ozone breaks the chlorine bonds, facilitating biodegradation of the resulting compounds.
Enhanced Biodegradation
Combinations of anaerobic and aerobic biological degradation represent a strategy that is theoretically capable of completely degrading chlorinated solvents to harmless by-products.1 The strategy has been primarily applied to the dissolved phase of chlorinated solvent plumes. A promising biotechnology for DNAPL removal is enhanced reductive dechlorination. Under highly anaerobic conditions, chlorinated solvents such as PCE, TCE, and DCE are used as electron acceptors by subsurface bacteria. In this process, chlorine atoms are sequentially removed from the chlorinated solvent molecule.
Natural attenuation processes refer to biodegradation, dispersion, sorption, and volatilization, all of which affect the fate and transport of chlorinated solvents in all hydrological systems. When these processes are shown to be capable of attaining site-specific remediation objectives in a time period that is reasonable compared to other strategies, they may be selected alone or in combination with other more active remedies as the preferred remedial strategy. The EPA defines monitored natural attenuation as the reliance on natural attenuation processes (within a controlled and monitored cleanup approach) to achieve site-specific remedial objectives within a time frame that is reasonable compared to other methods.8 Natural attenuation processes include biodegradation, dispersion, dilution, sorption, volatilization, and chemical or biological stabilization, transformation, or destruction of contaminants. Such processes are typically used in conjunction with other active remediation measures or as a follow-up these that have already been implemented.
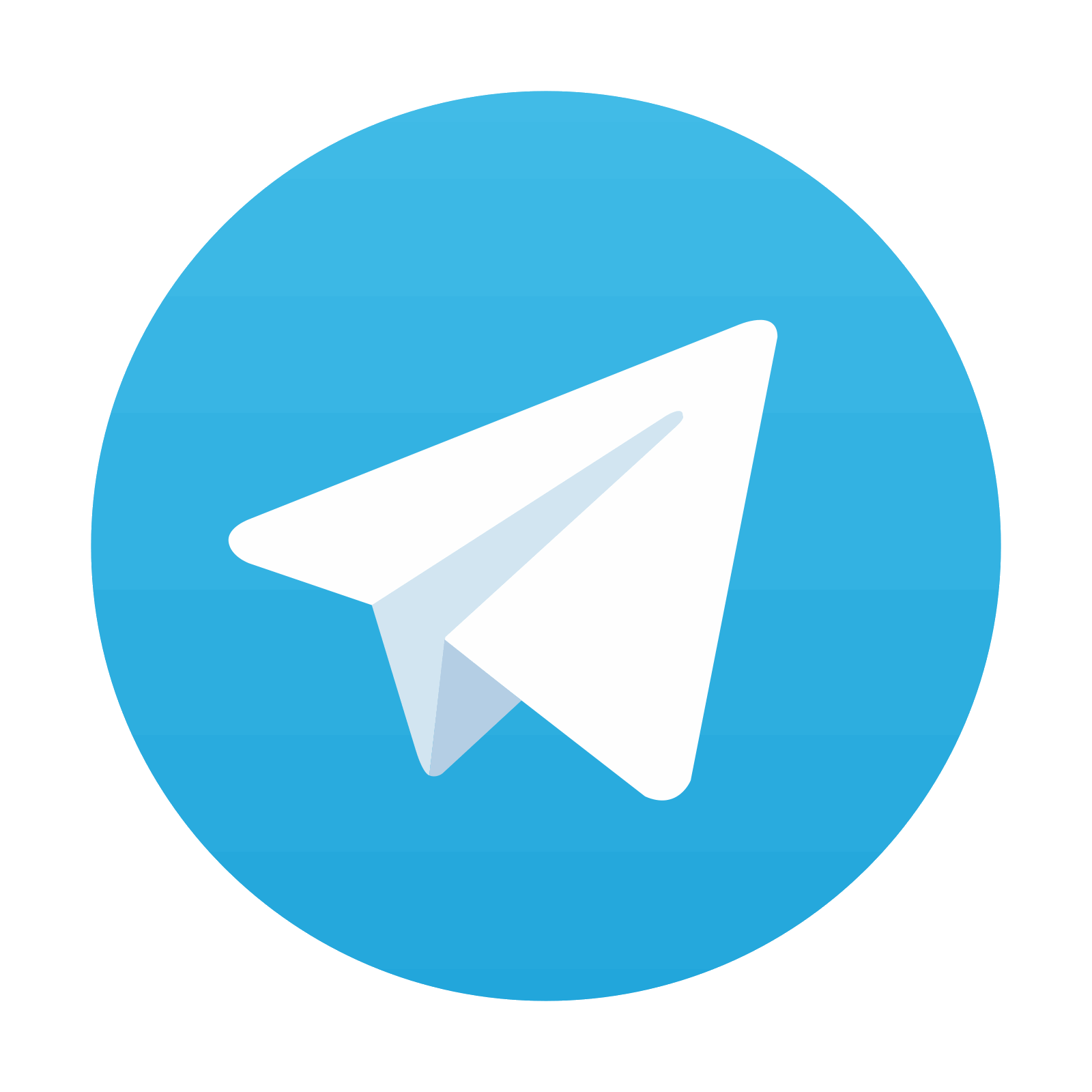
Stay updated, free articles. Join our Telegram channel
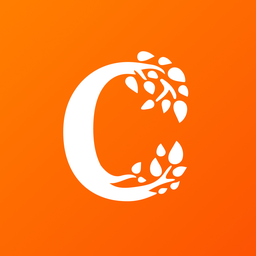
Full access? Get Clinical Tree
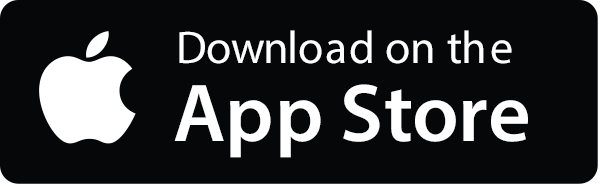
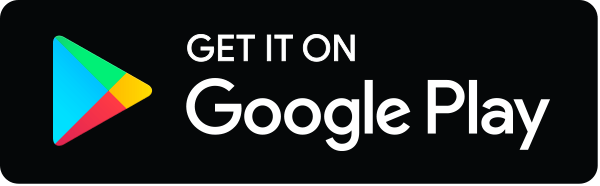