Chapter 21 Glycolysis, Tricarboxylic Acid Cycle, and Oxidative Phosphorylation
For the generation of metabolic energy, all major nutrients are degraded to acetyl coenzyme A (acetyl-CoA). These include carbohydrates, fat, protein, and alcohol (Fig. 21.1). Acetyl-CoA is also called “activated acetic acid” because it consists of an acetyl (acetic acid) group that is bound to coenzyme A by an energy-rich thioester bond.
This chapter shows how glucose is oxidized and how this process produces energy in the form of ATP. Glucose is not only the most abundant monosaccharide in food but is also produced from other monosaccharides, by the breakdown of the storage polysaccharide glycogen, and from amino acids and other noncarbohydrate substrates (Fig. 21.2).
Glucose uptake into the cells is regulated
Table 21.1 summarizes the most important facilitated-diffusion glucose carriers. One of these carriers, GLUT4, is insulin dependent. Its deposition in the plasma membrane is enhanced by insulin (Fig. 21.3). The consequence is that muscle and adipose tissue take up glucose after a carbohydrate-rich meal, when the insulin level is high, but not during fasting, when the insulin level is low. Muscle and adipose tissue do not depend on glucose but can subsist on fatty acids and other nutrients if needed. During fasting, glucose is redirected from muscle and adipose tissue to tissues that depend on glucose, including brain and erythrocytes.
Table 21.1 Most Important Glucose Transporters
Transporter | Expressed in | Function |
---|---|---|
GLUT1 | Most tissues | Basal glucose uptake |
GLUT2 | Liver, intestine, pancreatic β-cells | High-capacity glucose uptake |
GLUT3 | Brain | Neuronal glucose uptake |
GLUT4 | Muscle, adipose tissue, heart | Insulin-dependent glucose uptake |
GLUT5 | Intestine | Fructose transport |
Glucose degradation begins in the cytoplasm and ends in the mitochondria
The steps in glucose oxidation are summarized in Figure 21.4. The initial reaction sequence, known as glycolysis, is cytoplasmic. It turns one molecule of glucose (six carbons) into two molecules of the three-carbon compound pyruvate. All cells of the body are capable of glycolysis.
In these pathways, the hydrogen of the substrate is transferred to the coenzymes NAD+ and FAD (see Chapter 5). The reduced coenzymes donate electrons to the respiratory chain of the inner mitochondrial membrane, which in turn donates them to molecular oxygen. The reoxidation of the reduced coenzymes is highly exergonic. It is the energy source for ATP synthesis by oxidative phosphorylation. The TCA cycle and oxidative phosphorylation take place in all cells that contain mitochondria.
Glycolysis begins with atp-dependent phosphorylations
After entering the cell, glucose is phosphorylated to glucose-6-phosphate by hexokinase (Fig. 21.5). The hexokinase reaction is irreversible for two reasons: Its ΔG0′ is strongly negative (−4.0 kcal/mol) because an energy-rich phosphoanhydride bond in ATP is cleaved while a “low-energy” phosphate ester bond is formed (Table 21.2), and the ATP concentration in a healthy cell is always far higher than the ADP concentration.
Table 21.2 Standard Free Energy Changes of Glycolytic Reactions
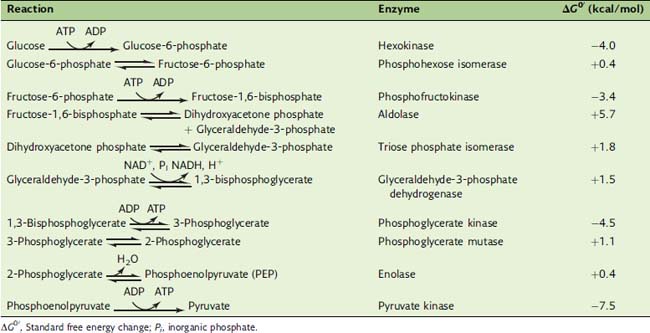
ΔG0′, Standard free energy change; Pi, inorganic phosphate.
Most glycolytic intermediates have three carbons
The last enzyme of glycolysis, pyruvate kinase, makes substrate-level phosphorylation by transferring the phosphate group of PEP to ADP. Although ATP is synthesized, this reaction is highly exergonic with a standard free energy change (ΔG0′) of −7.5 kcal/mol. This implies a free energy content of 14.8 kcal/mol for the phosphate ester bond in PEP. Why is this phosphate ester so unusually energy rich? The initial transfer of phosphate from PEP to ADP is indeed endergonic. However, the enolpyruvate formed in this reaction rearranges almost immediately to pyruvate. This highly exergonic reaction removes enolpyruvate from the equilibrium (Fig. 21.6).
Overall, the reactions of glycolysis produce a net yield of two ATP molecules and two NADH molecules for each molecule of glucose (Table 21.3).
Table 21.3 Products Formed during Conversion of One Molecule of Glucose to Two Molecules of Pyruvate in Aerobic Glycolysis*
Enzyme | Product (Molecules) |
---|---|
Hexokinase | −1 ATP |
Phosphofructokinase | −1 ATP |
Glyceraldehyde-3-phosphate dehydrogenase | +2 NADH |
Phosphoglycerate kinase | +2 ATP |
Pyruvate kinase | +2 ATP |
2 ATP + 2 NADH |
* Note that all reactions beyond the aldolase reaction occur twice for each glucose molecule.
Only the hexokinase, PFK, and pyruvate kinase reactions are “irreversible.” The aldolase and triose phosphate isomerase reactions have very unfavorable equilibria (see Table 21.2). Nevertheless they can proceed because fructose-1,6-bisphosphate is formed in the irreversible PFK reaction and glyceraldehyde-3-phosphate is rapidly consumed in the next reactions of the pathway. The actual equilibrium of the glyceraldehyde-3-phosphate dehydrogenase reaction is far more favorable than suggested by its ΔG0′ value of +1.5 kcal/mol because NAD+ is far more abundant than NADH in the aerobic cell.
Lactate is produced under anaerobic conditions
The solution to this problem is simple (Fig. 21.7). The enzyme lactate dehydrogenase (LDH) regenerates NAD+ by transferring the hydrogen of NADH to the keto group of pyruvate:
The overall balance of lactate formation by anaerobic glycolysis is
Another limitation is that the two ATP molecules formed in glycolysis capture only 14.6 kcal of useful energy, whereas the complete oxidation of glucose produces approximately 270 kcal (see Table 21.7). Therefore anaerobic glycolysis is useful only under certain circumstances, for example:
Table 21.7 Energy Yield from Glucose Oxidation
Pathway | Yield (Molecules) |
---|---|
Glycolysis | 2 ATP |
2 NADH → 4 or 6 ATP* | |
Pyruvate dehydrogenase | 2 NADH → 6 ATP |
Tricarboxylic acid cycle | 2 GTP → 2 ATP |
6 NADH → 18 ATP | |
2 FADH2 → 4 ATP | |
36 or 38 ATP |
⁎ The energy yield from cytoplasmic NADH depends on the shuttle system used.
CLINICAL EXAMPLE 21.3: Lactic Acidosis
Some other causes of lactic acidosis are listed in Table 21.4. In alcohol intoxication, for example, the rapid formation of NADH during alcohol oxidation increases the [NADH]/[NAD+] ratio. This makes the liver unable to oxidize lactate, and lactate is released into the blood.
Table 21.4 Conditions Resulting in Lactic Acidosis
Condition | Mechanism |
---|---|
Physical exercise | Anaerobic glycolysis in muscle |
Severe lung disease High altitude Drowning ![]() | Impaired respiration |
Severe anemia Carbon monoxide poisoning Sickling crisis ![]() | Impaired oxygen delivery |
Cyanide poisoning | Inhibition of oxidative phosphorylation |
Alcohol intoxication von Gierke disease ![]() | Elevated [NADH]/[NAD+] ratio Impaired gluconeogenesis |
Pyruvate dehydrogenase deficiency | Impaired pyruvate oxidation |
Leukemia Metastatic carcinoma ![]() | Anaerobic glycolysis by neoplastic cells |
Pyruvate is decarboxylated to acetyl-CoA in the mitochondria
where CoA-SH = uncombined CoA. This irreversible reaction is catalyzed by pyruvate dehydrogenase, a multienzyme complex with three components:
The structures of thiamin pyrophosphate (TPP) and lipoic acid are shown in Figure 21.8. TPP acts as a carrier of pyruvate and of the hydroxyethyl group that is formed by pyruvate decarboxylation. Lipoic acid participates as a redox system and carrier of the acetyl group. The reaction sequence is shown in Figure 21.9.
The TCA cycle produces two molecules of carbon dioxide for each acetyl residue
In the first reaction, the acetyl group of acetyl-CoA reacts with the four-carbon compound oxaloacetate to form the six-carbon compound citrate. This irreversible reaction (Table 21.5) is catalyzed by citrate synthase. The remaining reactions regenerate oxaloacetate from citrate, with two carbons released as carbon dioxide (Fig. 21.12).
Table 21.5 Standard Free Energy Changes (ΔG0′) of Pyruvate Dehydrogenase Reaction and Tricarboxylic Acid Cycle Reactions
Enzyme | ΔG0′ (kcal/mol) | Products |
---|---|---|
Pyruvate dehydrogenase | −8.0 | CO2, NADH |
Citrate synthase | −8.5 | |
Aconitase | +1.6 | |
Isocitrate dehydrogenase | −2.0 | CO2, NADH |
α-Ketoglutarate | −8.0 | CO2, NADH |
dehydrogenase | ||
Succinyl-CoA synthetase | −0.7 | GTP |
Succinate dehydrogenase | ≈0 | FADH2 |
Fumarase | −0.9 | |
Malate dehydrogenase | +7.1 | NADH |
CLINICAL EXAMPLE 21.4: Arsenic Poisoning
Arsenic occurs in two forms that are toxic by different mechanisms. Arsenate is a structural analog of phosphate that competes with phosphate in many biochemical reactions. However, the bonds that arsenate forms with phosphate and carboxyl groups are unstable and hydrolyze spontaneously. Figure 21.10 shows how it uncouples substrate-level phosphorylation in glycolysis. The term “uncoupling” implies that the pathway can proceed, but without ATP synthesis.
Arsenite is even more toxic than arsenate. It poisons pyruvate dehydrogenase and other lipoic acid containing enzymes by binding to the sulfhydryl groups in dihydrolipoic acid (Fig. 21.11). A similar reaction of arsenite with closely spaced sulfhydryl groups in immature keratin leads to its incorporation in hair and fingernails. Its determination in hair is used forensically in cases of alleged arsenic poisoning.
Citrate is isomerized to isocitrate by aconitase. The enzyme first dehydrates citrate to aconitate and then hydrates aconitate to isocitrate (Fig. 21.13). At equilibrium, the composition is 90% citrate, 3% aconitate, and 7% isocitrate.
Fluoroacetate has occasionally been used as a rat poison but is interesting for terrorists as well. It is metabolically converted to fluorocitrate by the same enzymes that otherwise metabolize acetate (Fig. 21.14). The resulting fluorocitrate is a potent inhibitor of aconitase.
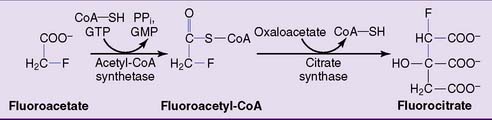
Figure 21.14 Metabolic activation of fluoroacetate to fluorocitrate. Fluorocitrate is an inhibitor of aconitase.
Isocitrate is oxidatively decarboxylated to α-ketoglutarate (2-oxoglutarate) by isocitrate dehydrogenase. Oxalosuccinate is an enzyme-bound intermediate in this reaction (Fig. 21.15). The isocitrate dehydrogenase of the TCA cycle is an NAD-linked enzyme.
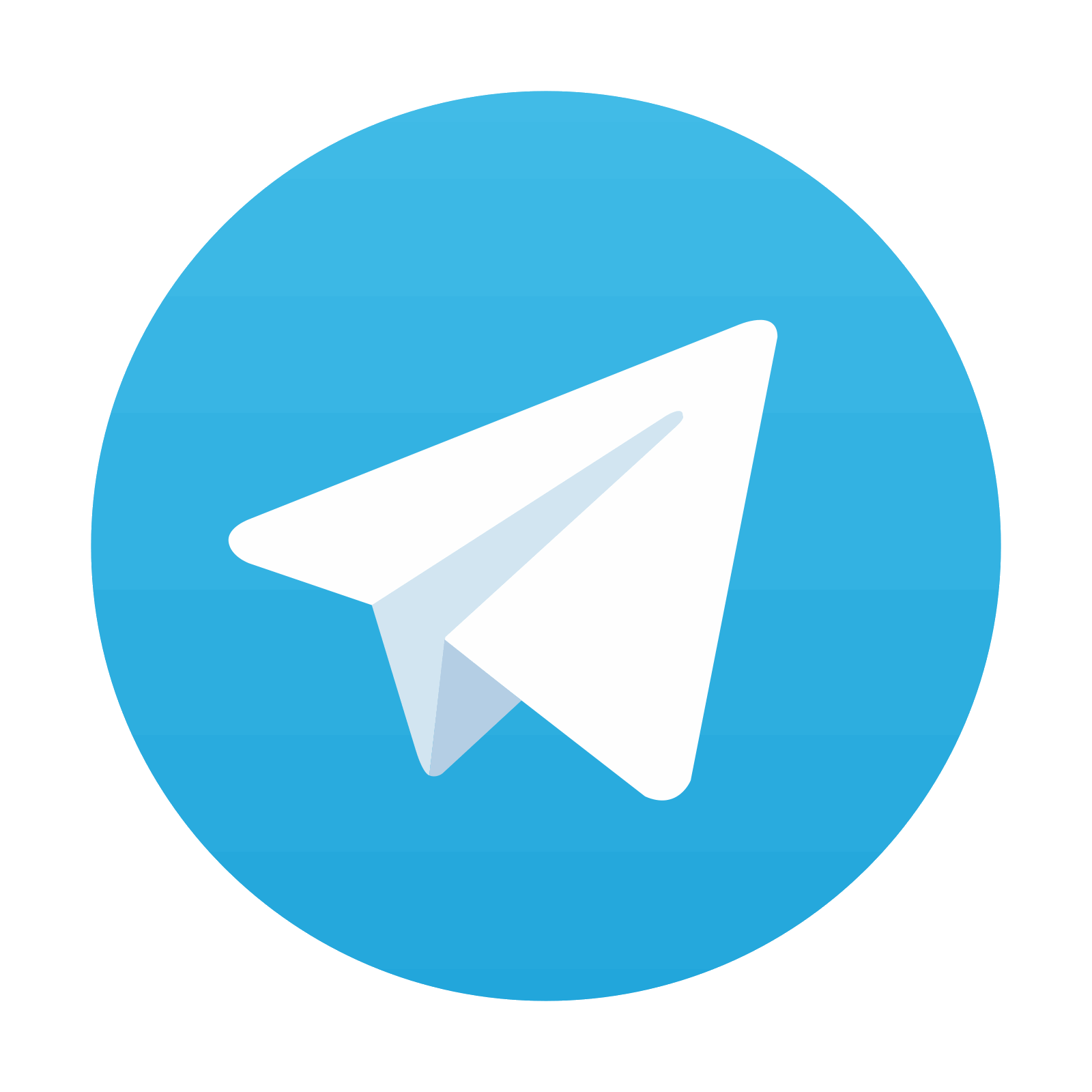
Stay updated, free articles. Join our Telegram channel
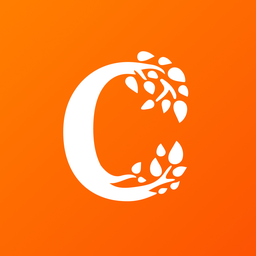
Full access? Get Clinical Tree
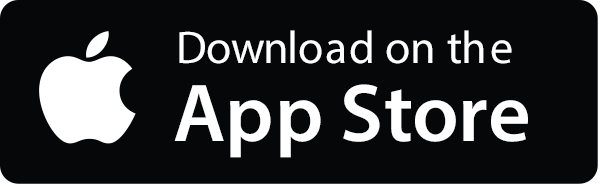
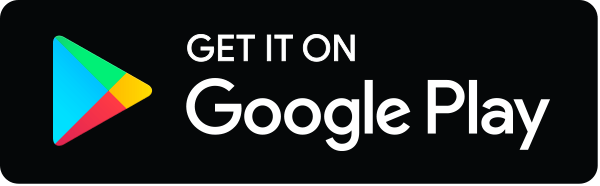