Scientific, technological, and medical advances have made it possible to detect, diagnose, and treat most common diseases (e.g., asthma, diabetes, and hypertension) early in their course and more effectively than ever before. However, such advances are highly dependent on the skills and knowledge of clinicians, access to health-care services, and the availability and affordability of diagnostic technologies. Most health-care providers follow a conventional model in which a patient presents with a set of symptoms and signs, which the provider uses to make a “most likely” diagnosis. The provider then prescribes a treatment that they think will be most effective. If this treatment fails, the process is repeated until a correct diagnosis or more effective treatment is found. In such a model, preventive health maintenance is encouraged. Compliance, however, is challenging, because information about risk factors, as well as the patient’s perception of risk is at best approximate.
Precision or predictive medicine is a model of practice in which each person’s personal risk for rare and common conditions and the effectiveness of various treatments are estimated directly from their unique combination of genetic and environmental risk factors. Accordingly, a health-care provider can predict a person’s risk for common diseases, select diagnostic tests to confirm the presence of disease, and prescribe the best therapeutic regimen to treat it. Ideally, knowledge of disease risk promotes interventions (e.g., modification of diet, choice of drug therapy) that not only can treat disease early in its course, but also can delay its onset or prevent it altogether.
The effectiveness of precision medicine depends on a number of factors. These include identifying genetic and environmental risk factors (and their interactions) that enable accurate prediction of clinically significant risk; demonstrating that individual risk assessment improves diagnostic accuracy and treatment outcome; developing technologies for cost-efficient assessment of a person’s genome; building an infrastructure for clinicians to access risk data, interpret risk information, and explain risk estimates to patients; and developing guidelines and policies for how risk assessment information should be used in clinical and research applications. Not all of these aims will be achieved for every common disease. Indeed, for many complex diseases, it is likely that there will be no alternative to the conventional model of practice in the near future because so little is known about their etiology and pathophysiology. Nevertheless, for some common diseases and drug responses, genetic testing, and in several cases, precision medicine, are already being adapted to the clinical setting.
In this chapter, we discuss how new technologies are making the assessment of individual human genomes widely accessible, how such genome-wide information is being used currently to make personal decisions about health, and the implications of precision care.
Precision medicine is the use of each person’s unique combination of genetic and environmental risk factors to make predictions about their disease risk and response to various treatments.
A Technology-Driven Transformation
Traditionally the search for genetic variants that influence risk for Mendelian conditions and common complex diseases has been a daunting task and has been one of the major obstacles to developing precision medicine. The most common approach to finding such variants involved testing whether polymorphisms in candidate genes were associated with disease risk in a small group of unrelated patients with the same phenotype (e.g., diabetes, obesity). This was problematic, in part because choosing the most appropriate candidate genes was difficult, small cohorts provided limited statistical power, and the process of genotyping or sequencing was labor intensive and expensive. This situation changed dramatically with the development of technologies to interrogate millions of polymorphisms per person cheaply and efficiently ( Box 14.1 ) and more recently the introduction of inexpensive exome and genome sequencing (see Chapter 8 ). These technologies, coupled with advances in statistics and computing, enabled the application of new approaches such as genome-wide association studies (see Chapter 8 ), as well as the study of much larger cohorts of tens or hundreds of thousands of persons. In addition, these new technologies for genotyping and DNA sequencing have made it possible to develop cost-effective clinical tests that take advantage of newly discovered risk variants. The major challenge nowadays to implementing precision medicine is translating discoveries of risk variants and their roles in biological pathways into clinical practice.
Knowledge of a person’s genetic makeup will clearly be an important tool for making better decisions about health, medical care, and perhaps lifestyle as well. Until recently, assessing the genome as a whole was fairly expensive and done only in research laboratories. However, new technologies have dramatically lowered the cost of whole-genome analysis and have spurred the development of consumer services that offer genome-wide studies directly to the public, e.g. “direct-to-consumer” genotyping (see Chapter 13 ). These services have rapidly made headlines, as much for their novelty as for their potential to inform persons about their genetic composition.
Most whole-genome consumer services offer to genotype millions of common single nucleotide polymorphisms (SNPs). The SNPs typed for consumers are the same ones commonly being used by researchers to identify disease-gene associations for common multifactorial disorders such as hypertension, diabetes, and obesity. As gene-disease associations are reported, consumers who have access to their genetic information can evaluate their risk for genetic diseases. Also, because each person’s genetic data are permanent, evaluation of risk can be reassessed with each new discovery. However, many of the SNP-disease associations reported to consumers are relatively weak and may be misunderstood or misinterpreted by the lay consumer (see Chapter 13 ).
More recently exome sequencing and whole-genome sequencing have been made available to the public. This service is still expensive and therefore very limited in application. It remains unclear whether a person’s understanding of health-related risks will be increased by knowing about some or all of the genetic risk variants that they carry. Most of the caveats raised in the previous paragraph for whole-genome SNP typing apply equally to whole-genome sequencing. However, since risk variants for Mendelian conditions are frequently detected by sequencing, some variants of potential clinical utility are virtually always found.
The Impact of Genomics
Pharmacogenetics
Many of the drinks (e.g., coffee, tea) and foods that we ingest each day contain thousands of complex compounds that each of us must process. Some of these compounds never leave the gastrointestinal tract, but most are absorbed, distributed, metabolized, and eliminated (i.e., biotransformed) to a variety of products that are used immediately, stored, or excreted. Exogenously synthesized compounds that are administered to achieve a specific effect on the human body (e.g., pharmaceuticals) also undergo biotransformation, and humans vary in the efficiency and speed with which they do this. Moreover, the response of a drug’s target (e.g., enzymes, receptors) can also vary among individuals. The study of the individual genetic variants that modify human responses to pharmacological agents is called pharmacogenetics; the assessment of the action of many genes simultaneously is called pharmacogenomics.
Genetic Prediction of Serious Adverse Drug Responses
Over the last decade ambitious efforts have been undertaken to advance the knowledge of pharmacogenetics. This has been driven, in part, by the expectation that through the use of pharmacogenetics, we will be able to profile DNA differences among individuals and thereby predict responses to different medicines. For example, a genetic profile (i.e., a summary of a person’s risk alleles) might predict who is more or less likely to respond to a drug or to suffer a serious adverse drug reaction (SADR).
Many drugs have a response rate between 25% and 75%. For example, angiotensin-converting-enzyme (ACE) inhibitors and beta blockers have been found to be ineffective or only partially effective in up to 70% of hypertensive patients. The use of such drugs in persons who are unlikely to respond increases the incidence of SADRs and adds to the burden of health-care costs. Yet, for most drugs, no tests are available to determine who will or will not respond, so these drugs are administered largely on a trial-and-error basis.
Many drugs have adverse effects that are of clinical importance, and of the approximately 1200 drugs approved for use in the United States, about 15% are associated with a significant incidence of SADRs. A widely cited analysis conducted in the mid-1990s suggested that nearly two million people are hospitalized each year as a result of adverse drug effects, and approximately 100,000 people die from them, even when the drugs are appropriately prescribed and administered. Studies in Europe and Australia have yielded similar results. Thus identification of genetic profiles that predict a person’s response to drugs is likely to increase the overall efficacy and safety of pharmaceuticals.
Testing is currently available for hundreds of alleles that predict SADRs. For example, thiopurine methyl transferase (TPMT) is an enzyme that inactivates thiopurine drugs (e.g., 6-mercaptopurine, azathioprine), which are frequently used to treat acute lymphatic leukemia and to prevent rejection of organ transplants. A mutation of the TPMT gene reduces enzyme activity. About one in 300 persons of European ancestry is homozygous for this mutation, and these patients can experience life-threatening bone marrow suppression upon exposure to thiopurine drugs. The presence of such variants can be assessed by genotyping or by enzyme assays, which are now commonly done before administering thiopurines. Large-scale exome sequencing studies have demonstrated that each person carries at least several alleles that alter drug metabolism compared to the general population.
Each person’s response to natural and synthetic chemicals is determined in part by polymorphisms in genes that control pathways of biotransformation and the chemical’s target.
Individualized Drug Therapy
One of the major challenges of pharmacogenetics is the selection of appropriate targets (e.g., a specific enzyme, cytokine, or cell-surface receptor) that might be amenable to manipulation by a drug. The results of genetic studies are used to identify polymorphisms associated with varying susceptibility to disease (i.e., a potential target for a drug) or polymorphisms that modify the human response to a drug. For example, the drug, ivacaftor, selectively improves the activity of cystic fibrosis (CF) transmembrane receptor (CFTR) channel activity, and therefore lung function in a subset of persons with mutations that diminish CFTR activity (see Clinical Commentary 4.1). Long QT syndrome (LQT syndrome; see Chapter 12 ) can be caused by mutations in one of at least fifteen different genes whose protein products affect ion channel (e.g., potassium, sodium and calcium channels) function in heart cells. For example, because sodium channels and calcium channels are blocked by different drugs, a person’s genetic profile can be used to choose the best drug for treatment of LQT syndrome. Similarly, genetic forms of epilepsy can be caused by different types of ion channels, and thus are treated by different types of drugs. In each of these cases, the relationship between disease and target is well characterized, facilitating individualized drug treatment.
Polymorphisms in genes that encode angiotensinogen, angiotensin-converting enzyme, and the angiotensin II type 1 receptor have been associated with differential responses to antihypertensive agents. For example, the ACE gene contains a 190-bp sequence that can be either present (the I allele) or deleted (the D allele). Persons who are homozygous for the D allele are more responsive to ACE inhibitors. Response to antihypertensive beta blockers has been associated with polymorphisms in genes that encode subunits of the β-adrenergic receptor ( Table 14.1 ). None of these variants are commonly tested prior to initiating antihypertensive therapy, but studies are under way to determine when such information, in conjunction with environmental risk factors such as smoking and diet, might facilitate the development of individualized treatment.
Gene | Enzyme/Target | Drug | Clinical Response |
---|---|---|---|
CYP2D6 | Cytochrome P4502D6 | Codeine | Persons homozygous for an inactivating mutation do not metabolize codeine to morphine and thus experience no analgesic effect |
CYP2C9 | Cytochrome P4502C9 | Warfarin | Persons heterozygous for a polymorphism need a lower dose of warfarin to maintain anticoagulation |
VKORC1 | Vitamin K epoxide reductase | Warfarin | Persons heterozygous for a polymorphism need a lower dose of warfarin complex, subunit 1, to maintain anticoagulation |
NAT2 | N -acetyltransacetylase 2 | Isoniazid | Persons homozygous for slow-acetylation polymorphisms are more susceptible to isoniazid toxicity |
TPMT | Thiopurine S-methyltransferase | Azathioprine | Persons homozygous for an inactivating mutation develop severe toxicity if treated with standard doses of azathioprine |
ADRB2 | β-Adrenergic receptor | Albuterol | Persons homozygous for a polymorphism get worse with regular use of albuterol |
KCNE2 | Potassium channel, voltage-gated | Clarithromycin | Persons heterozygous for a polymorphism are more susceptible to life-threatening arrhythmias |
SUR1 | Sulfonylurea receptor 1 | Sulfonylureas | Persons heterozygous for polymorphisms exhibit diminished sensitivity to sulfonylurea-stimulated insulin secretion |
F5 | Coagulation factor V (Leiden) | Oral contraceptives | Persons heterozygous for a polymorphism are at increased risk for venous thrombosis |
Many of the physiological effects of variation in drug response have been known for decades. A deficiency of glucose-6-phosphate dehydrogenase (G6PD), which is estimated to affect more than 200 million people worldwide, causes increased sensitivity to the antimalarial drug, primaquine, producing an acute hemolytic anemia. The metabolism of isoniazid (a drug commonly used to treat tuberculosis) is strongly influenced by an allele of the gene that encodes N -acetyltransacetylase 2 (NAT2), the enzyme that is used to acetylate, and thereby inactivate, isoniazid. Persons who are homozygous for this allele are known as slow inactivators and are at higher risk for developing side effects than persons who metabolize isoniazid more quickly. About half of persons of European or African ancestry are slow inactivators, but this figure is lower among East Asians. Succinylcholine is a drug widely used in anesthesia to induce short-term muscle paralysis. Typically, the effects of succinylcholine last only a few minutes before it is rapidly degraded in the plasma by circulating butyrylcholinesterase. Several alleles of the gene that encodes butyrylcholinesterase cause reduced enzyme activity. Persons who are homozygotes or compound heterozygotes for such alleles have a diminished ability to inactivate succinylcholine. This can result in prolonged paralysis and respiratory failure that requires mechanical ventilation for up to several hours.
In each of these examples, a person who has a relatively common allele might, upon exposure to a specific chemical, experience an unanticipated pharmacological effect. Variants have been discovered in enzymes that produce a much broader effect on the body’s response to multiple drugs. An example is debrisoquine hydroxylase, an enzyme encoded by the gene CYP2D6. This gene is a member of the cytochrome P450 superfamily, which encodes many different enzymes responsible for the biotransformation of compounds with widely divergent chemical structures. Polymorphisms of CYP2D6 affect the metabolism of more than 25% of all pharmaceuticals, including β-adrenergic receptor antagonists, neuroleptics, and tricyclic antidepressants ( Fig. 14.1 ). Another example is variants in CYP2C19 that diminish the rate at which the prodrug clopidogrel, an antiplatelet drug, is metabolized to its active form. Clopidogrel is commonly used in the more than two million persons who undergo stenting of their coronary arteries, and persons with a variant in CYP2C19 that reduces function are at substantially higher risk of stent thrombosis, which can precipitate a heart attack and death. All of these are examples of relatively simply genetic profiles (i.e., single polymorphisms) that affect drug response. Many drug responses are likely to be determined by much more complex profiles that are composed of multiple polymorphisms at multiple loci.
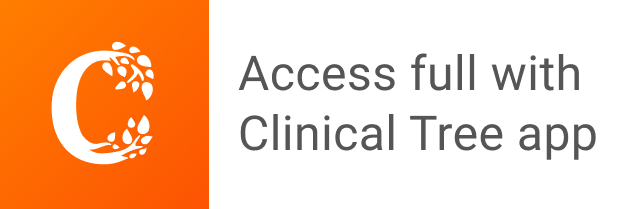