1 Robert C. James, Stephen M. Roberts, and Phillip L. Williams The intent of this chapter is to provide a concise description of the basic principles of toxicology and to illustrate how these principles are used to make reasonable judgments about the potential health hazards and the risks associated with chemical exposures. This chapter explains: The literal meaning of the term toxicology is “the study of poisons.” The root word toxic entered the English language around 1655 from the Late Latin word toxicus (which meant poisonous), itself derived from toxikón, an ancient Greek term for poisons into which arrows were dipped. The early history of toxicology focused on the understanding and uses of different poisons, and perhaps even today most people tend to think of a chemical or products labeled as a “toxic” substance” as that group of chemicals for which minimal exposure inevitably leads to death or some serious long-term adverse effect like cancer. As toxicology has evolved into a modern science it has expanded to encompass all forms of adverse health effects that any substance might produce. The following definitions are provided to help the reader understand several basic terms that may be used in this and other chapters: As these definitions indicate, the toxic responses that form the study of toxicology span a broad biological and physiological spectrum. Effects of interest may range from something relatively minor such as irritation or tearing to a more serious response like acute and reversible liver or kidney damage, to an even more serious and permanent disability such as cirrhosis of the liver or liver cancer. Given this broad range of potentially adverse effects to consider, it is perhaps useful for those unfamiliar with toxicology to define some additional terms, listed in order of relevance to topics that will be discussed in Chapters 2–24 of this book. Toxicology has become a science that builds on and uses knowledge developed in many related medical sciences, such as physiology, biochemistry, pathology, pharmacology, medicine, and epidemiology, to name only a few. Toxicology has evolved from the study of poisons to the study of all adverse effects induced by all chemicals or substances. Although toxicology is a science where a number of areas of specialization have evolved, all toxicologists fall into three principal areas of endeavor: descriptive toxicology, research/mechanistic toxicology, and applied toxicology. Descriptive toxicologists are scientists whose work focuses on the toxicity testing of chemicals. This work is done primarily at commercial and governmental toxicity testing laboratories, and the studies performed at these facilities are designed to generate basic toxicity information that identifies the various organ toxicities (hazards) the test agent is capable of inducing over those exposure conditions necessary to induce each effect. A thorough description of a chemical’s toxicology would identify all possible acute and chronic toxicities, including the genotoxic, reproductive, teratogenic (developmental), and carcinogenic potential of the test agent. It would identify important metabolites of the chemical that are generated as the body attempts to break down and eliminate the chemical, as well as understand how the chemical is absorbed into the body and distributed to tissues throughout the body, identify tissue accumulation or elimination, and ultimately determine how it is excreted from the body. Hopefully, appropriate dose–response test data are generated for those toxicities of greatest concern and that toxicity produced at the lowest dose during the completion of the descriptive studies so that the relative safety of any given exposure or dose level that humans might typically encounter can be predicted. Basic research or mechanistic toxicologists are scientists who study the chemical or agent in depth for the purpose of gaining an understanding of how the chemical or agent initiates those biochemical or physiological changes within the cell or tissue that result in the toxicity (adverse effect). The goal of mechanistic studies is to understand the specific biological reactions (i.e., the adverse chain of events) within the affected organism that ultimately result in the toxic effect being studied. Mechanistic experiments are performed at the molecular, biochemical, cellular, and tissue level of the affected organism. So, mechanistic assessments may incorporate and apply the knowledge of a number of many other related scientific disciplines within the biological and medical sciences (e.g., physiology, biochemistry, genetics, molecular biology, pathology). Because animal species are generally used to identify chemical-induced hazards, and because there may be significant species-specific responses to a chemical, mechanistic studies help provide the information on those key changes required to induce toxicity, and help reduce the uncertainty of the animal-to-human extrapolation we need to make to develop a safe exposure guideline. Applied toxicologists are scientists concerned with the use of chemicals in a “real world” or nonlaboratory setting. The primary goal of applied toxicologists is the control of chemical exposures in all work and nonwork environments by setting safe exposure guidelines for each exposure pathway (e.g., air, skin, ingestion exposure to the chemical) in that environment. Toxicologists who work in this area of toxicology use descriptive and mechanistic toxicity studies to limit the dose received by each or all exposure pathways to a total dose of the chemical that is believed to be safe. The process whereby this safe dose or level of exposure is derived is generally referred to as the area of risk assessment. Within applied toxicology a number of subspecialties occur. Forensic toxicology is that unique combination of analytical chemistry, pharmacology, and toxicology concerned with the medical and legal aspects of drugs and poisons; it is concerned with the determination of which chemicals are present and responsible in exposure situations of abuse, overdose, poisoning, and death that become of interest to the police, medical examiners, and coroners. Clinical toxicology specializes in ways to treat poisoned individuals and focuses on determining and understanding the toxic effects of medicines, simple over-the-counter (nonprescription) drugs, and other household products. Environmental toxicology is the subdiscipline concerned with those chemical exposure situations found in our general living environment. These exposures may stem from the agricultural application of chemicals, the release of chemicals during modern-day living (e.g., chemicals released by household products), regulated and unintentional industrial discharges into air or waterways, and various nonpoint emission sources (e.g., the combustion by-products of cars). Within this area there may be even further subspecialization (e.g., ecotoxicology, aquatic toxicology, mammalian toxicology, avian toxicology). Occupational toxicology is the subdiscipline concerned with the chemical exposures and diseases found in the workplace, the identification of the hazards or injuries that overexposure to an occupationally used chemical might represent, and the prevention of these exposures or the treatment of the injuries they might produce. Regardless of the specialization within toxicology, or the types of toxicities of major interest to the toxicologist, essentially every toxicologist performs one or both of the two basic functions of toxicology, which are to (1) examine the nature of the adverse effects produced by a chemical or physical agent (hazard/toxicity identification function) and (2) assess the probability of these toxicities occurring under specific conditions of exposure (dose–response and risk assessment function). Ultimately, the goal and basic purpose of toxicology is to understand the toxic properties of a chemical so that these adverse effects can be prevented by the development of appropriate handling or exposure guidelines. The hazard identification or the discovery of the toxicities a chemical produces requires the testing of chemicals at doses high enough to induce the full spectrum of toxicities a chemical can induce. Typically, the hazard identification process involves traditional animal testing to uncover the spectrum of adverse effects (hazards) the chemical is capable of producing at some dose. One way of characterizing and identifying the hazard is by examining toxicities as a function of exposure duration, as previously described for acute, subacute, subchronic, and chronic exposures. Because each chemical induces a different spectrum of toxic effects and one does not know beforehand which set of toxicity tests to perform to adequately capture and identify the possible hazards posed by the chemical, the chemical is examined using as wide a range of test systems as possible to ensure that all potential hazards for that chemical have been identified. For a complete toxicological evaluation the typical hazard assessment would follow a scheme similar to that illustrated in Figure 1.1. Typically, one would perform these tests using a tiered approach that starts with short exposure interval testing such as acute and subacute exposure periods (tier 1) and subsequently moves through subchronic tests (tier 2) and then chronic tests (tier 3). At each tier, specialized tests are performed in addition to those assessing target organ toxicities by route of exposure. For example, during the acute testing phase, dermal and reparatory tract irritation may be necessary as well as tests for the development of sensitization by the chemical. During subchronic and chronic testing, target organ testing is augmented by reproductive and developmental studies, testing for immunotoxicity, genotoxicity and mutagenicity, and a chronic bioassay for possible carcinogenic responses. Figure 1.1 A generic toxicity testing scheme that shows the ways in which a toxicity test might differ because of the different choices to be made regarding the duration of exposure, the route of exposure, or the endpoint to be measured in the study. A tiered approach such as this allows the dose ranges to be set and as the duration of exposure increases, the dose needed to induce the effect is usually lowered (see Table 1.1). The shorter the duration of exposure the lower the cost of the test and the more time-efficient the study. So, trying to identify the end points of interest and toxic dose range is done more time and cost efficiently by seeking the toxicities a chemical induces by testing the chemical short-term tests first. However, both the types of hazards seen and the doses inducing these effects can change with the duration of exposure; and the hazards seen at shorter exposure durations cannot be assumed to be those that will be found after longer durations of exposure. For example, cancer is a latent disease that may require a lifetime of exposure to detect. The route of exposure may also impact the hazard because as the site of absorption is altered it may impact the occurrence of localized effects (like irritation or cellular necrosis at the site of contact) and it can change the tissue distribution as well as the target organ concentration per unit of absorbed dose. Either change may produce a different pattern of target organs affected with different routes of exposure. For example, after testing trichloroethylene (TCE) for carcinogenicity using the mouse as the test organism, it was observed that inhalation exposure induced lung tumors but not liver tumors while oral administration induced liver tumors but not lung tumors. This kind of route-specific toxicity occurs frequently enough that regulatory agencies like the EPA no longer rely upon data gathered by one route of exposure to predict hazards or risk for another route of exposure, that is, there can be considerable uncertainty associated with route-to-route extrapolations without a mechanistic basis for doing so. Table 1.1 Examples Showing a NOAEL or LOAEL May Change with Exposure Duration Since we are looking for adverse outcomes, the primary source of information for hazard identification comes for toxicity tests using nonhuman species. Over the years, we have developed an extensive array of different toxicity test systems. These test systems are designed to examine end points of interest such as target organs, changes in physiological/biological/molecular function, the different chemical metabolites generated by enzymes whose function is the conversion of both endogenous and exogenous substances into chemical forms more easily eliminated from the body, the mechanism or modes of action, and chemical reactions with key cellular macromolecules (e.g., enzymes, proteins, RNA, DNA). For example, besides animal or whole organism test results, a toxicologist might use a specialized in vitro test system that involves test tube or cell culture methods to examine effects on cellular macromolecules, isolated cell fractions, cellular organelles (e.g., mitochondria), tissue fractions, and isolated perfused whole organs as procedures for examining specific molecular, physiological, or biological functions. A toxicologist might also perform in vivo tests in a variety of nonmammalian organisms ranging from simple, single cell organisms (e.g., bacteria, algae) to larger and more complex nonmammalian organisms like nematodes, fruit flies, Daphnia magna, or fish, particularly when attempting to identify the ecological hazards or an environmental pollutant. Some tests are easier and cheaper to perform and can better handle high-volume testing to screen candidate chemicals for further, more detailed toxicity testing or to predict toxicities in chemicals that have not been tested sufficiently via animal tests. One illustration of this approach is where toxicities are receptor-mediated and structure activity relationships may be used as a surrogate measure of subchronic and chronic hazards induced by structurally similar chemicals. The ever-expanding use of in vitro test systems may also be desirable in certain situations because they can isolate specific physiological or biochemical pathways in a way that better controls specific test conditions, doses, and outcomes besides being more time- and cost-efficient than whole organism testing. However, in vitro tests remove cell or target organism functions from the experimental in vitro concentrations (surrogate dose measure) used or the end point being measured may be modified in ways not easily extrapolated to whole organism responses. So, while in vitro tests may be undertaken more easily and repeated more consistently, they also have inherently greater uncertainty in comparison to what happens in a whole organism at specific exposure levels or exposure duration. For example, what metabolites are the chemical converted to in whole organisms that are not be seen when using certain in vitro test systems? Are toxic or nontoxic metabolites produced by the organism? How does the dose influence the metabolism and distribution throughout the body of the chemical and/or its metabolites? Are the exposure conditions of an in vitro system much higher than those that occur in tissues when the chemical is administered in whole animal experiments? In the end, in vivo or whole organism testing in a variety of species is generally necessary to identify the range of possible hazards the chemical might pose to humans. In addition to animal methods, hazard information associated with human exposure to the chemical may also be available. As discussed in more detail elsewhere, there can be significant species differences in the both the beneficial and adverse responses induced by a chemical. So, in the final hazard assessment for a chemical, a toxicologist would like to review as much human data as are available. There are four basic categories of epidemiological information that can assist the hazard evaluation. These categories are occupational epidemiology (mortality and morbidity studies), clinical exposure studies, accidental acute poisonings, and chronic environmental epidemiology studies. The advantages and disadvantages of the hazard information typically provided by these four categories of human toxicological information and that of traditional in vitro and animal toxicity tests are summarized and compared in Table 1.2. Table 1.2 Some of the Advantages and Disadvantages of Toxicity Data by Category It is probably safe to say that among lay individuals there exists considerable confusion about the term toxic. If asked, most lay individuals would probably define a toxic substance using either a definition that one would apply to highly poisonous or very potently toxic chemicals or something that implies that only some chemicals produce adverse effects in humans and so can be described as toxic chemicals or those substances that we should all avoid. To help illustrate this point, and to begin to emphasize the fact that the toxicity is a function of dose, the reader is invited to take the following pop quiz. First, cross-match the doses shown in column A that produce lethality in 50% of the animals (lethal dose [LD50]) with the chemicals listed in column B. These chemicals are a collection of food additives, medicines, drugs of abuse, poisons, pesticides, and hazardous substances for which the correct LD50 is listed somewhere in column A. To perform this cross-matching, first photocopy Table 1.3 and simply mark the ranking of the dose (i.e., the number corresponding next to the dose in column A) you believe correctly corresponds to the chemical it has been measured for in column B. (Note: The doses are listed in descending order, and the chemicals have been listed alphabetically. So, the three chemicals you believe to be the safest should have the three largest doses [you should rank them as 1, 2, and 3], and the more unsafe or dangerous you perceive the chemical to be, the higher the numerical ranking you should give it. After testing yourself with the chemicals listed in Tables 1.3, review the correct answers in tables found at the end of this chapter.) Table 1.3 Cross-Matching Exercise: Comparative Acutely Lethal Doses Answers to Table 1.3A Comparative Acutely Lethal Doses According to the ranking scheme that you selected for these chemicals, were the least potent chemicals common table salt, vitamin K (which is required for normal blood clotting times), the iron supplement dosage added to vitamins for individuals that might be slightly anemic, or a common pain relief medication you can buy at a local drugstore? What were the three most potentially toxic chemicals (most dangerous at the lowest single dose) in your opinion? Were they “natural” or the “synthetic” (human-made) chemicals? How toxic did you rate the nicotine that provides the stimulant properties of tobacco products? How did the potency ranking of prescription medicines like the sedative phenobarbital or the pain killer morphine compare to the acutely lethal potency of a poison such as strychnine or the pesticide malathion? Now, take the allowable workplace chronic exposure levels for the following chemicals—aspirin, gasoline, iodine, several different organic solvents, and vegetable oil mists—and again rank these substances going from the highest to lowest allowable workplace air concentration (listed in Table 1.4). Remember that the lower (numerically) the allowable air concentration, the more potently toxic the substance is per unit of exposure. Review the correct answers for tables recreated at the end of this chapter. Table 1.4 Cross-Matching Exercise: Occupational Exposure Limits—Aspirin and Vegetable Oil Versus Industrial Solvents Answers to Table 1.4 Occupational Exposure Limits: Aspirin and Vegetable Oil Versus Industrial Solvents
GENERAL PRINCIPLES OF TOXICOLOGY
1.1 BASIC DEFINITIONS AND TERMINOLOGY
1.2 TOXICOLOGY: A DIVERSE SCIENCE WITH TWO BASIC GOALS
1.3 HAZARD IDENTIFICATION FUNCTION
Exposure Duration
Species (Strain)
Organ/End Point
Dose (mg/kg/day)
a. NOAEL Comparisons
1,4-Dioxane
Acute (2 weeks)
Rat (Fischer-344)
Hepatic
1040
Intermediate (13 weeks)
60
Chronic (2 years)
16
Acute (2 weeks)
Rat (Fischer-344)
Renal
1040
Intermediate (13 weeks)
330
Chronic (2 years)
21
Di(2-ethylhexyl)phthalate
Acute (once)
Rat (Fischer-344)
Renal
5000
Intermediate (90 days)
Rat (Wistar)
1900
Chronic (1 year)
Rat (Sherman)
200
b. LOAEL Comparisons
1,4-Dioxane
Acute (2 weeks)
Rat (Fischer-344)
Hepatic
2750
Intermediate (13 weeks)
150
Chronic (2 years)
81
Acute (2 weeks)
Rat (Fischer-344)
Renal
2750
Intermediate (13 weeks)
760
Chronic (2 years)
103
Di(2-ethylhexyl)phthalate
Acute (7 days)
Rat (Wistar)
Hepatic
2000
Intermediate (21 days)
1730
Chronic (79 weeks)
1000
Advantages
Disadvantages
a. Occupational Epidemiology (Human) Studies
May have relevant exposure conditions for the intended use of the chemical.
Exposures (especially past exposures) may have been poorly documented.
As these exposure levels are usually far higher than those found in the general environment, even low or frank effect levels may allow for a realistic extrapolation of a safe level for environmental exposures.
Difficult to properly control; many potential confounding influences (lifestyle, concurrent diseases, genetic, etc.) are inherent to most work populations. These potential confounders are often difficult to identify.
The chance to study the interactive effects of other chemicals that might be present. Again at high doses relative to most environmental situations.
Post facto—not necessarily designed to be protective of health.
Separating interactive effects resulting from combinations of chemical exposures may be difficult or impossible.
Avoid uncertainties inherent in extrapolating toxicities and dose–response relationships across species.
The increase in disease incidence may have to be large or the measured response severe to be able to demonstrate the existence of the effect being monitored (e.g., cancer). The power to detect risk may be limited.
The full range of human susceptibility (sensitivity) may be measurable if large enough, and diverse enough, populations can be examined.
The full range of human sensitivity for the toxicity of interest may not be measurable because some potentially sensitive populations (young, elderly, infirm) are not represented.
May help identify gender, race, or genetically controlled differences in responses.
Effects must be confirmed by multiple studies as heterogeneous populations are examined and confounders cannot always be excluded.
The potential to study human effects is inherent to almost all industrial uses of chemicals. Thus, a large number of different possible exposure/chemical regimens are available to study.
Often costly and time-consuming. Cost–benefit may be low if confounders or other factors limit the range of exposures, toxicities, confounders, or population variations that might occur with the chemical’s toxicity.
b. Clinical (Human) Exposure Studies
The toxicities identified and the dose–response relationship measured are reported for the most relevant species to study (humans).
The most sensitive group (e.g., young, elderly, infirm) may often be inappropriate for study.
Typically, the components of these studies are better defined and controlled than occupational epidemiology studies. Prospective study design, rather than retrospective design, is used.
Moderately costly to costly to perform.
The chance to study the interactive effects of other chemicals.
Usually limited to shorter exposure intervals than epidemiological studies.
The dose–response relationship is measured in humans. Exposure conditions may be altered during the exposure interval in response to the presence or lack of an effect making NOAELs or LOAELs easier to obtain.
Only NOAELs are targeted for study. These studies are primarily limited to examining safe exposure levels or effects of minimal severity. More serious effects caused by the chemical cannot intentionally be examined by this type of study.
Better than occupational studies for detecting relatively subtle effects. Greater chance to control for the many confounding factors that might be found in occupational studies.
Chronic effects are generally not identifiable by this type of study.
Allows the investigator to test for and identify possible confounders or potential treatments.
Requires study participant compliance.
Allows one to test the specific subpopulations of interest.
May require confirmation by another study.
May help identify gender, race, or genetically controlled differences in responses.
May raise ethical questions about intentionally exposing humans to toxicants.
May be the best method for allowing initial human exposure to the chemical, particularly if medical monitoring is a prominent feature of the study.
Unexpected human toxicities may occur as animal extrapolations are not perfect.
Use of randomization improves the study design and provides best causal inference.
The change being monitored may be statistically significant but still of unknown biological/clinical relevance, leaving the interpretation of results open to question.
c. Environmentally Exposed Epidemiological Studies
The toxicities identified and the dose–response relationship measured are reported for the most relevant species to study (humans).
Exposures to the chemical are typically low relative to other types of human exposures to the chemical in question, or to chemicals causing related toxicities (e.g., exposure to other environmental carcinogens). Thus, attributing the effects observed in a large population may be difficult if many confounding risk factors are present and uncontrolled for in the exposed population.
Exposure conditions are relevant to understanding or preventing significant environmentally caused health effects from occurring.
The exposure of interest may be so low that it is nontoxic and only acting as a surrogate indicator for another risk factor that is present but not identified by the study.
The chance to study the effects of interactive chemicals may be possible.
The number of chemicals with interactive effects may be numerous and their exposures large relative to the chemical of interest. This will confound interpretations of the data.
The full range of human susceptibility may be present.
The full range of human susceptibility may not be present.
May allow one to test specific subpopulations of interest for differences in thresholds, response rates, and other important features of the dose–response relationship.
The full complement of relevant environmental exposure that is associated with the population are not necessarily identified or considered.
May help identify gender, race, or genetically controlled differences in responses.
Large populations may be so heterogeneous in their makeup that when compared to control responses that differences in confounders, gender, age, race, and so on, may weaken the ability to discriminate real disease associations of the chemical exposure from other causes of the disease.
There may be too many potential confounders to identify and control for and the correlation may be coordinated rather than causal, that is, the problem of the ecological fallacy.
Exposures are frequently not quantified at the individual level.
d. Acute Accidental Poisonings
Exposure conditions are realistic for this particular safety extrapolation. In most instances, poisonings are limited to acute exposure situations.
Because the exposure is either accidental or related to a suicide attempt, accurate exposure/dose information is frequently lacking.
These studies often provide a temporal description indicating how the disease will develop in an exposed individual.
This knowledge gained from these studies may be of limited relevance to all other human exposure situations.
Identifies the target organs affected by high, acute exposures. These organs may become candidate targets for chronic toxicity studies.
Confounding factors affecting the magnitude of the response may be difficult to identify as exposure conditions will not be recreated to identify modifying factors.
The clinical response requires no planning as the information gathering typically consists of responding to and treating the organ injuries present as they develop.
Acute toxicities may not mimic those seen with chronic exposure. This may mislead efforts to characterize the effects seen under chronic exposure situations.
These studies are typically case reports or a small case series and so measures of individual variations in response may be difficult to estimate.
These chance observations develop without warning, a feature that prevents the development of a systematic study by interested scientists who are knowledgeable about the chemical.
Because these typically occur as emergency situations, important clinical data may not always be collected.
e. Animal Toxicity Tests
Easily manipulated and controlled.
Test species response is of uncertain human relevance. Thus, the predictive value is lower than that of human studies.
Best ability to measure subtle responses.
Species/strain/sex/age responses may vary significantly both qualitatively and quantitatively. Thus, a number of different species/strains (both sexes) should ideally be tested.
Widest range of potential toxicities to study.
Exposures levels may not be relevant to (they may far exceed) the human exposure level. The restricted environment of the animal study may not be representative of the complex and variable environment of humans. For example, the practice of allowing animals to eat at will (ad libitum feeding) in bioassays has been shown to increase response rates of certain carcinogens.
Chance to identify and elucidate mechanisms of toxicity that allow for more accurate risk extrapolations to be made using all five categories of toxicity test data.
Selecting the best animal species to study, that is, the species with the most accurate surrogate responses, is always unknown and is difficult to determine a priori (without a certain amount of human test data). Thus, animal data poses somewhat of a Catch-22 situation, that is, you are testing animals to predict human responses to the chemical but must know the human response to that chemical to accurately select the proper animal test species. Mechanisms that are developed may be unique to that species/strain/sex being tested.
Cheaper to perform than full-scale epidemiology studies.
May be a poor measure of the variability inherent to human exposures because animal studies are so well controlled for genetics, doses, observation periods, and so on.
No risk of producing adverse human health effects during the study.
The reproducibility of the animal response may create a false sense of precision when attempting human extrapolations.
f. Alternatives to Traditional Animal Testing
Type of Toxicity Test
Advantages
Disadvantages
Structure–activity relationships (SARs)
Does not require the use of any experimental animals.
Many toxicants with very similar chemical properties have very different toxicities.
Quick to perform.
In vitro testing
Reduces the number of experimental animals needed.
Cannot fully approximate the complexities that take place in whole organisms (i.e., absorption, distribution, biotransformation, and elimination).
Allows for better control of the toxicant concentration at the target site.
Allows for the study of isolated functions such as nerve–muscle interaction and release of neurotransmitter.
Easier to control for host factors such as age dependency, nutritional status, and concurrent disease.
Possible to use human tissue.
Alternative animal testing (nonmammalian and nonavian species)
Less expensive and quicker (due to shorter lifespans) than using higher animals.
Since the animal is far removed from humans, the effect of a toxicant can be very different from that found with higher animals.
Since a whole organism is used it allows for absorption, distribution, biotransformation, and elimination of the toxicant.
1.4 DOSE–RESPONSE/RISK ASSESSMENT FUNCTION
The chemicals listed in this table are not correctly matched with their acute median lethal doses (LD50’s). Rearrange the list so that they correctly match. The correct order can be found in the answer table at the end of the chapter.
A
B
N
LD50 (mg/kg)
Toxic Chemical
Correct Order
1
15,000
Alcohol (ethanol)
___________
2
10,000
Arrow poison (curare)
___________
3
4,000
Dioxin or 2,3,7,8-TCDD
___________
4
1,500
(PCBs)—an electrical insulation fluid
___________
5
1,375
Food poison (botulinum toxin)
___________
6
900
Iron supplement (ferrous sulfate)
___________
7
150
Morphine
___________
8
142
Nicotine
___________
9
2
Insecticide (malathion)
___________
10
1
Rat poison (strychnine)
___________
11
0.5
Sedative/sleep aid (phenobarbitol)
___________
12
0.001
Tylenol (acetaminophen)
___________
13
0.00001
Table salt (sodium chloride)
___________
Actual Ranking No.
LD50 (mg/kg)
Toxic Chemical
1
15,000
PCBs
2
10,000
Alcohol (ethanol)
3
4,000
Table salt—sodium chloride
4
1,500
Ferrous sulfate—an iron supplement
5
1,375
Malathion—a pesticide
6
900
Morphine
7
150
Phenobarbitol—a sedative
8
142
Tylenol (acetaminophen)
9
2
Strychnine—a rat poison
10
1
Nicotine
11
0.5
Curare—an arrow poison
12
0.001
2,3,7,8-TCDD (dioxin)
13
0.00001
Botulinum toxin (food poison)
The chemicals listed in this table are not correctly matched with their allowable workplace exposure levels. Rearrange the list so that they correctly match. The correct order can be found in the answer table at the end of the chapter.
N
Allowable Workplace Exposure Level (mg/m3)
Chemical (Use)
Correct Order
1
0.05
Aspirin (pain reliever)
___________
2
5
Gasoline (fuel)
___________
3
10
Iodine (antiseptic)
___________
4
54
Perchloroethylene (dry-cleaning fluid)
___________
5
55
Tetrahydrofuran (organic solvent)
___________
6
75
Trichloroethylene (solvent/degreaser)
___________
7
147
1,1,1-Trichloroethane (solvent/degreaser)
___________
8
170
1,1,2-Trichloroethane (solvent/degreaser)
___________
9
890
Toluene (organic solvent)
___________
10
1910
Vegetable oil mists (cooking oil)
___________
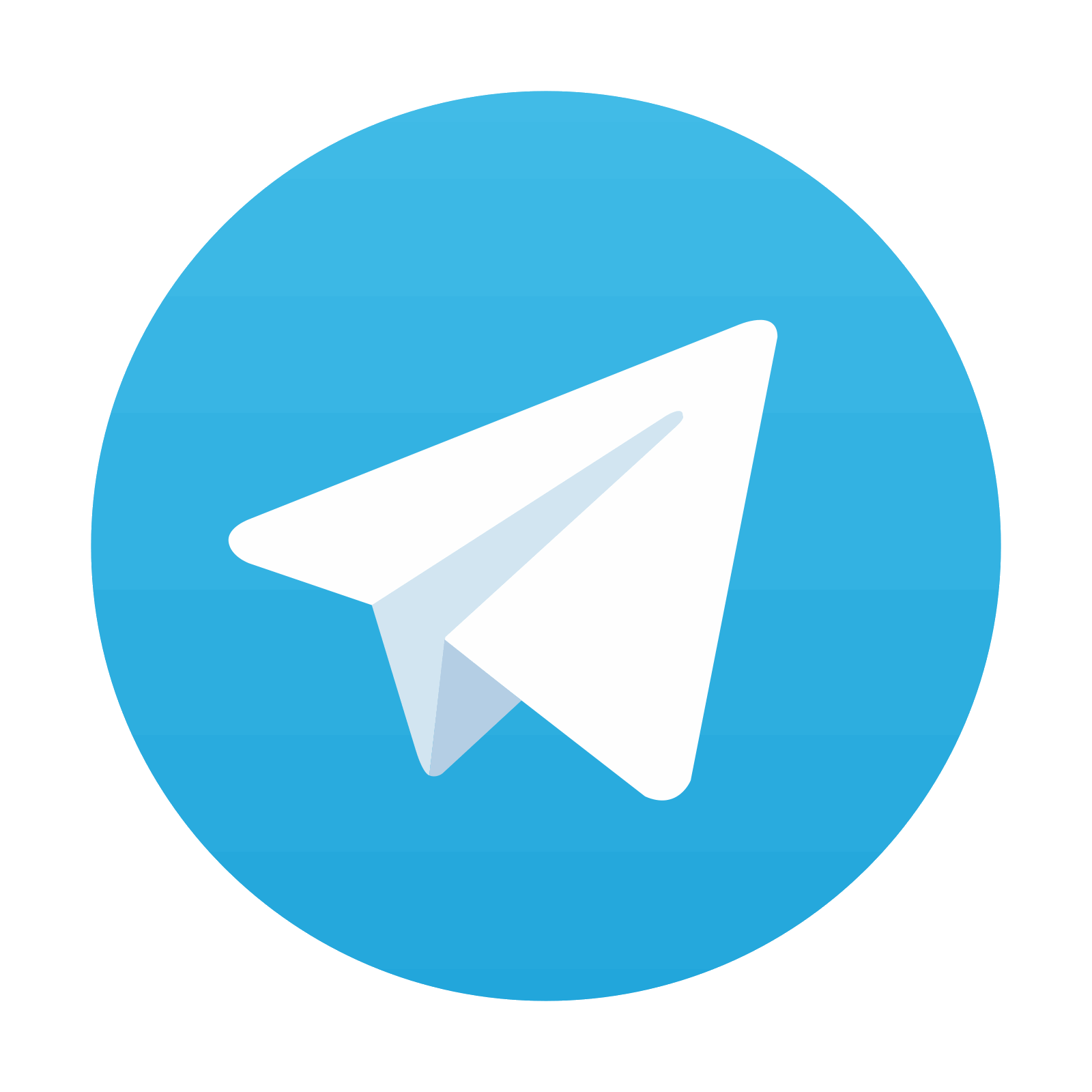
Stay updated, free articles. Join our Telegram channel
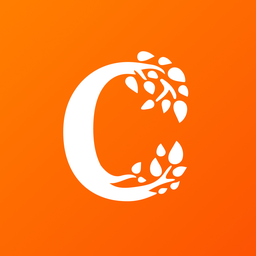
Full access? Get Clinical Tree
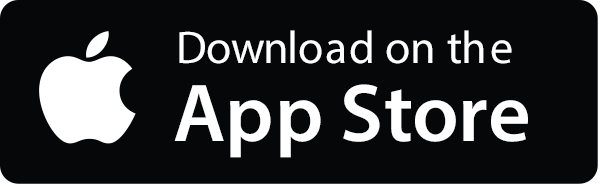
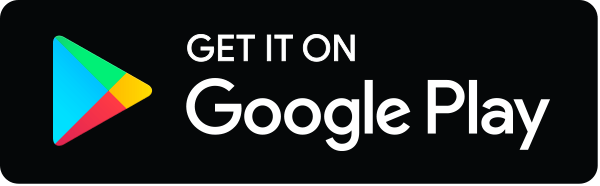