Introduction
Gastrointestinal (GI) diseases most often present with one or more of four common classes of symptoms and signs: (1) abdominal or chest pain; (2) altered ingestion of food (eg, resulting from nausea, vomiting, dysphagia [difficulty swallowing], odynophagia [painful swallowing], or anorexia [lack of appetite]); (3) altered bowel movements (ie, diarrhea or constipation); and (4) GI tract bleeding, either occurring without warning or preceded by one or more of the foregoing (Table 13–1). However, not all cases of a particular GI disease present in the same way. For example, peptic ulcer disease, although typically accompanied by abdominal pain, may be painless.
Cardinal GI Symptom or Sign | Esophagus | Stomach | Intestines | Gallbladder |
---|---|---|---|---|
Pain | Achalasia, reflux | Gastric ulcer | Duodenal ulcer | Cholelithiasis |
Gastric cancer | Irritable bowel syndrome | |||
Diverticular disease | ||||
Altered ingestion | ||||
Dysphagia | Achalasia, reflux | |||
Nausea, vomiting | Achalasia, reflux | Gastroparesis | Acute gastroenteritis | Cholelithiasis |
Esophageal cancer | Obstruction | |||
Altered bowel movements | ||||
Constipation | Diverticular disease | |||
Diabetic autonomic neuropathy | ||||
Diarrhea (including steatorrhea) | Gastric surgery, dumping syndrome | Gastroenteritis | Cholelithiasis | |
Irritable bowel syndrome | ||||
Inflammatory bowel disease | ||||
Diabetic autonomic neuropathy | ||||
Bleeding | ||||
Hematemesis | Varices resulting from portal hypertension | Gastric ulcer | Duodenal ulcer | |
Mucosal laceration (eg, after violent retching) | ||||
Bloody stools (including melena, frank blood, and occult blood) | Varices | Gastric ulcer | Inflammatory bowel disease Duodenal ulcer | |
Diverticular disease | ||||
Colon cancer | ||||
Gastroenteritis | ||||
Infarction |
GI disease may be limited to the GI tract (eg, reflux esophagitis, peptic ulcer, diverticular disease), be a manifestation of a systemic disorder (eg, inflammatory bowel disease), or present as a systemic disease resulting from a primary GI pathologic process (eg, vitamin deficiencies resulting from malabsorption). Because different parts of the GI tract are specialized for certain functions, the most prominent causes, consequences, and manifestations of disease differ from one anatomic site to another.
Acutely, GI disease can be complicated by dehydration, sepsis, or bleeding or by their consequences, such as shock. Dehydration can occur as a consequence of even subtle alterations in fluid input or outflow because the volume of fluid traversing the GI tract daily is enormous (see later discussion). Sepsis can result from disruption of the barrier function against pathogens in the environment, including bacteria resident in the colon. The tendency for bleeding is a reflection of the tremendous vascularity of the GI tract and the difficulty of applying pressure at the site of bleeding.
Chronically, GI disease can be complicated by malnutrition and deficiency states. These occur because many primary GI diseases result in malabsorption (failure to absorb one or more necessary nutrients in ingested food).
GI tract disease can present as partial or complete obstruction (blockage of movement of contents down the GI tract) caused by adhesions and stenosis resulting from proliferation of connective tissue in response to inflammation. The symptoms and signs of obstruction can range from mild nausea, abdominal pain, and anorexia to projectile vomiting and rebound tenderness. In severe cases, obstruction can result in perforation, infarction and bleeding, hypotension, shock, sepsis, and death. The severity of symptoms depends on the extent of obstruction, the degree to which the obstruction compromises blood flow to the affected region, and the stage in the natural history of the process at which the patient presents for medical attention.
Checkpoint
1. What are the cardinal symptoms and signs of GI disease?
2. What are some acute systemic complications of primary GI disease?
3. What additional systemic manifestations can occur as a result of chronic GI disease?
Structure, Function, & Control of the GI Tract
The GI tract is one of the most complex and important organ systems. It comprises the alimentary canal, a hollow structure extending from the mouth to the anus, and associated glandular organs (salivary glands, pancreas, gallbladder, and liver) that empty their contents into the canal (Figure 13–1). The GI tract, which is 7–9 m in the adult, includes the mouth, esophagus (23–25 cm), stomach, small intestine (duodenum, jejunum, ileum; 6–7 m), large intestine (cecum and colon; 1.0–1.5 m), rectum, and anus. The GI tract is connected to the salivary glands, the pancreas, and the gallbladder, the sources of exocrine secretions that play an essential role in digestion.
The wall of the GI tract is composed of four main layers. From the lumen outward, these include the mucosa, submucosa, muscularis externa, and serosa (Figure 13–2). The precise structure of some of these layers, most notably the mucosa, varies from one region of the GI tract to the next. The mucosa has three components: specialized epithelial cells that line the lumen; the underlying lamina propria, a layer of connective tissue that contains small blood and lymphatic vessels, immune cells and nerve fibers; and the muscularis mucosa, a thin layer of muscle cells. The muscularis mucosa is an important boundary in determining whether cancer of the GI tract is still localized to its site of origin or is likely to have metastasized (ie, spread to distant regions of the body). The submucosa is a layer of loose connective tissue directly beneath the mucosa containing larger blood and lymphatic vessels and a nerve plexus of the intrinsic or enteric nervous system, termed the submucosal nerve (Meissner) plexus. This nerve plexus is particularly important for control of secretion in the GI tract. In some areas, the submucosa also contains glands and lymphoid tissue. The muscularis externa is composed of an inner circular and an outer longitudinal layer of smooth muscle and is responsible for motility of the GI tract. Between these muscle layers lies the myenteric nerve (Auerbach) plexus, a division of the enteric nervous system that regulates motility. The serosa is an outer sheath of squamous mesothelial cells and connective tissues, where larger nerves and blood vessels travel in a bed of connective and adipose tissue.
The overall function of the GI tract is to take in nutrients and process them to a form that can be used by the body and to eliminate wastes. The major physiological processes that occur in the GI tract are digestion, secretion, motility, and absorption.
Food is taken into the mouth as large particles containing macromolecules that are not immediately absorbable into the body. Digestion is the process that converts nutrients in food to products that can be absorbed by cells of the mucosa. Digestion includes physical processes (eg, chewing, GI contractions) that break up the food, mix it with digestive secretions, and propel it along the alimentary canal, and chemical processes (eg, digestive enzymes) that degrade food components (proteins, fats, polysaccharides) to products that can be absorbed (amino acids, fatty acids, monosaccharides). Digestive enzymes arise from exocrine glands (salivary gland, pancreas, gallbladder, and liver) and from cells and glands in the mucosa or are found on the apical surface of certain epithelial cells.
During the process of digestion, large volumes of fluid are secreted into the lumen of the GI tract. Secretions arise from exocrine glands (salivary glands, pancreas, gallbladder) and from epithelial cells lining the GI lumen (or glands that connect to the lumen). The daily fluid load in the GI tract is approximately 2 L of oral intake and 7 L of secretions (1.5 L saliva, 2.5 L gastric juice, 0.5 L bile, 1.5 L pancreatic juice, and 1 L intestinal secretions). From this total of 9 L, approximately 100 mL ends up in stool daily; the balance is recycled (Figure 13–3).
Secretions and luminal contents are moved from mouth to anus and mixed by a process termed motility, because of the coordinated contractions of smooth muscle. Smooth muscle cells have a resting membrane potential (small excess of negative charge) in their interior as a result of the activity of pumps in the plasma membrane. When a cell is depolarized, this potential difference is transiently abolished, generating a signal that (1) causes contraction of actin and myosin filaments and (2) is propagated to neighboring cells, resulting in the coordinated response of muscle contraction. Depolarization of a cell can occur spontaneously or in response to a neural or hormonal stimulus depending on the specific characteristics of different cells. GI smooth muscle displays differences in contractile properties in different regions of the tract. “Slow-wave” oscillating depolarizations occur in some areas and rapid “spike” depolarizations in other areas. Each type occurs with a characteristic intrinsic frequency, but each can also be triggered by specific stimuli such as stretch, neuronal input, or hormones. Short bursts of spikes cause phasic motor activity; longer bursts cause tonic muscle contraction. Tonic contraction occurs at sphincters (“gates” that allow further movement down the GI tract only during relaxation). Phasic electrical activity occurs at the intervening regions of the GI tract (between sphincters).
The products of digestion (amino acids, small peptides, monosaccharides, fatty acids) are taken into the body by the process of absorption. Absorbed molecules can pass across (transcellular route) or between (paracellular route) the epithelial cells lining the intestine to enter the blood or lymphatic systems. In general, this transport can occur by either a passive, energy-independent mechanism that occurs down an electrochemical gradient (of charge or concentration), or by an active, energy-requiring process that occurs against an electrochemical gradient. Passive transport can occur by simple diffusion (random molecular motion) of uncharged molecules that readily pass the lipid layer plasma membrane. In this manner, short-chain fatty acids are absorbed in the small intestine. Charged molecules that cannot cross the plasma membrane diffuse through specialized channels (transmembrane proteins) within the apical and basolateral membrane of epithelial cells. For instance, water is absorbed by diffusion through aquaporins (proteins that form water channels) in the small intestine. Some molecules that are absorbed by diffusion bind to transporter proteins in the plasma membrane that facilitate their transfer into the cell (facilitated diffusion). For example, fructose is absorbed into epithelial cells of the small intestine by facilitated diffusion through the apical membrane GLUT-5 transporter.
Active transport requires metabolic energy. There are two classes of active transport. In primary active transport, the transport molecule itself hydrolyzes adenosine triphosphate (ATP). An example of primary active transport is the Na-K ATPase found in the basolateral membrane of intestinal epithelial cells, which expels three Na+ ions from cells in exchange for two K+ ions that are pumped into the cell. This unequal transport of ions generates a transmembrane potential (negative inside; ie, transport is electrogenic). In secondary active transport, the transporter itself does not hydrolyze ATP, but transport depends on an electrochemical gradient that has been established by primary active transport. The Na-K ATPase maintains a low intracellular Na+ concentration and an inside negative potential in epithelial cells, thereby providing the electrochemical gradient for secondary active transport of many absorbed molecules. For example, glucose is absorbed against a concentration gradient across the apical membrane of epithelial cells in the small intestine by secondary active transport with Na+ ions by the SGLT1 transporter. Two Na+ ions are transported down their electrochemical gradient (generated by the Na-K ATPase), dragging with them one glucose molecule. For large molecules such as proteins, transport occurs by pinching off from, and fusion of membrane vesicles with, the plasma membrane. These processes are termed endocytosis (uptake into epithelial cells) and exocytosis (export out of epithelial cells).
In addition to the major roles of the GI tract that are related to digestion and absorption, the digestive tract has other functions that are essential for maintenance of health and homeostasis.
The mucosa of the GI tract is the largest surface of the body that is exposed to the environment, and the gut, like the skin, must protect the body from the external environment. Defense involves protection against ingested toxins, bacteria, and viruses, as well as the bacteria and toxins that normally exist in the large intestine (Table 13–2). The magnitude of the problem is illustrated by the observation that there are more bacterial cells in the human colon than cells in the entire body. Defense involves two mechanisms.
Forms of Defense | Structural Adaptations | Functional Adaptations | Mechanism of Defense |
---|---|---|---|
Defense from acid | |||
Mucus production | Large numbers of mucus-secreting stomach surface cells | Mucin gene expression | Prevents direct contact of acid with epithelium |
Bicarbonate production (alkaline tide) | Duodenal Brunner glands | Neutralizes any acid that breaches epithelium | |
Prostaglandin production | Specialized prostaglandin-producing cells in lamina propria | Cyclo-oxygenase 1 and 2 (COX1/2) gene expression | Attenuates acid production |
Tight junctions | Tight junction formation | Prevents breach of epithelium | |
Bicarbonate from pancreas | Pancreatic duct opening into duodenum | Response of secretin to gastric acid | Neutralizes acid leaving stomach |
Defense from infection | |||
Secretory immune system | Mucosa-associated lymphoid tissue and transcytotic epithelial cells | Machinery for transcytosis of immunoglobulin | Extends to GI tract lumen the protective umbrella of blood-borne immunity |
Rapid epithelial cell turnover | Cell proliferation in glands/crypts; cell release into lumen | Limits the consequences of enterocyte infection | |
Normal colonic microbiota | Induce expression of specific antimicrobial proteins (angiogenin4, Reg3γ) | ||
Stomach acid | Gastric glands containing parietal cells | Multiple humoral controls on acid secretion (histamine, acetylcholine, and gastrin) | Kills pathogenic organisms on ingestion |
Adaptive immune defense—The mucosal immune system or gut-associated lymphoid tissue (GALT) surveys the contents of the intestinal lumen through a variety of mechanisms that utilize cells of both myeloid and lymphoid lineages. Myeloid-derived cells (specific populations of dendritic cells and macrophages) extend processes through the intestinal epithelial barrier that sense the luminal environment. Aggregates of lymphoid cells include Peyer patches (larger aggregates in the distal small intestine) and isolated lymphoid follicles located throughout the intestine. These lymphoid aggregates also play important roles in immune surveillance (Figure 13–4). GALT protects against pathogenic bacteria, viruses, and toxins and enables tolerance to potentially immunogenic dietary substances and bacteria.
Innate immune defense—These mechanisms include secretion of fluid (eg, abundant acid secreted by the stomach), electrolytes, and mucus, as well as the tight junctions between epithelial cells. The secretions neutralize and flush away potentially damaging bacteria and macromolecules, and the tight junctions of the gut epithelium prevent their ingress into tissues.
In the intestine, mucus is secreted by specialized goblet cells. The mucus forms a protective layer over the epithelial cells. A group of antimicrobial peptides are secreted into the intestinal lumen. The specialized cells in the small intestine that perform this function are the Paneth cells that produce and secrete lysozyme and alpha-defensins that contribute to defense and healing. Alpha-defensins have broad-spectrum activity and are thought to create holes in bacterial cell walls and prevent them from colonizing the small intestine. Trefoil peptides are secreted into the lumen of the GI tract with mucus. Among their many effects, they promote healing of mucosal lesions.
The small intestine receives 8–9 L of fluid with electrolytes per day and secretes a further 1 L and electrolytes per day. Most of the fluid is absorbed. Thus, secretion and absorption must be regulated to maintain balance. Increased secretion or diminished absorption causes diarrhea, which can be fatal because of fluid and electrolytes loss.
Undigested food products, bacteria, and certain heavy metals (eg, copper and iron excreted in bile) are excreted in feces.
Checkpoint
4. What are the major functions of the GI tract?
5. Describe the four major layers of a cross-section through the GI tract.
6. What volumes of fluid are transferred into and out of the GI tract each day?
7. Describe the general mechanism of electrolyte transport across epithelial cells.
8. Describe the defense mechanism of the GI tract.
The processes of motility, secretion, digestion, and absorption are under close physiologic regulation by nerves, hormones, and paracrine substance (Figure 13–5).
There are two components of GI innervation.
Intrinsic innervation by the enteric nervous system—The enteric nervous system is the third division of the autonomic nervous system (Figure 13–6). An enteric neuron has its cell body within the wall of the GI tract and is thus intrinsic to the gut. The enteric nervous system comprises a series of ganglionated nerve plexuses that extend from the esophagus to the rectum, which are organized into two principal components: 1) the myenteric, or Auerbach, plexus, which is sandwiched between the layers of the muscularis externa; and 2) the submucosal or Meissner plexus, which lies in the submucosa. The enteric nervous system is extensive, containing as many neurons as are present in the spinal cord. It contains sensory or afferent neurons (sometimes called intrinsic primary afferent neurons [IPANs]) that sense the environment (eg, intestinal pH, osmolality, wall stretch), interneurons (the connectors), and secretomotor or efferent neurons that control many cell types to stimulate or inhibit motility, secretion, absorption, and immune function of the GI tract. In this manner, the enteric nervous system can regulate the GI tract in a reflex manner without input from the CNS. For this reason, it is often called the “little brain.” Enteric neurons use many neurotransmitters, most notably neuropeptides.
The degree to which the CNS regulates the enteric nervous system varies with region. The characteristic functions of structures derived from the embryonic foregut (eg, esophageal peristalsis, relaxation of the lower esophageal sphincter, gastric accommodation and peristalsis, pyloric sphincter function) are more dependent on CNS control. However, functions of structures derived from the embryonic midgut and hindgut (eg, intestinal peristalsis and mucosal secretion) can continue without input from the CNS.
The clinical importance of the enteric nervous system is seen in clinical syndromes in which its function is lost, which can occur at several levels. In esophageal achalasia, for example, as a result of enteric nervous system defects, the body of the esophagus is quiet and the lower sphincter is tonically contracted, making ingestion of food difficult or impossible. More distally, failure of the enteric nerves to migrate into the colon during development (in Hirschsprung disease) or loss of enteric nervous system function (in pseudo-obstruction of the small bowel) have severe clinical consequences, including abdominal pain, distension, and a risk of catastrophic intestinal perforation.
Extrinsic innervation by parasympathetic and sympathetic nerves—Extrinsic neurons that innervate the GI tract have cell bodies outside of the gut wall and allow a bidirectional communication between the brain and the gut (the brain-gut axis) (Figure 13–7). This communication can regulate the function of the enteric nervous system or directly control the activity of other cell types.
Figure 13–6
The enteric nervous system. Left: Enteric nervous system of the small intestine shows that enteric neurons are organized in two nerve plexuses, the submucosal plexus and myenteric plexus, with other plexuses including the deep muscular, periglandular, and villous plexus. (Redrawn with permission from Costa M et al. Histochemistry of the enteric nervous system. In: Johnson LR, ed. Physiology of the Gastrointestinal Tract, 2nd ed. Raven Press, 1987.) Right: The enteric nervous system includes sensory neurons, interneurons, and motor neurons. Complete reflex arcs exist within the enteric nervous system.
Figure 13–7
The extrinsic innervation of the GI tract by the parasympathetic and sympathetic nerves. Preganglionic parasympathetic nerves from the medulla and the sacral spinal cord project fibers in the vagal and pelvic nerves, respectively, to the wall of the GI tract and innervate enteric neurons that serve as postganglionic parasympathetic nerves. Preganglionic sympathetic nerves project fibers from the thoracolumbar regions of the spinal cord to the prevertebral ganglia, where they innervate postganglionic sympathetic nerves that project to the GI tract. Both the parasympathetic and sympathetic preganglionic nerves release acetylcholine (ACh), which activates nicotinic receptors on postganglionic nerves. Postganglionic parasympathetic nerves release acetylcholine and peptides, whereas postganglionic sympathetic nerves release norepinephrine (NE).
In parasympathetic innervation, the vagus nerve (cranial nerve X) innervates the esophagus, stomach, gallbladder, pancreas, and the first part of intestine, cecum, and proximal colon. The pelvic nerve from the sacral spinal cord innervates the distal colon and the rectum. Preganglionic cell bodies in the medulla (vagus) or sacral spinal cord (pelvic nerve) project fibers to some enteric neurons in the gut wall, which are thus in a sense postganglionic parasympathetic nerves. The preganglionic nerves use acetylcholine as a neurotransmitter, which activates nicotinic receptors on enteric neurons. The postganglionic enteric nerves use acetylcholine (acting on muscarinic receptors) and neuropeptides as neurotransmitters. Parasympathetic stimulation can stimulate and inhibit GI functions.
In sympathetic innervation, preganglionic sympathetic nerves arise from cell bodies in the thoracic spinal cord and project fibers to prevertebral ganglia (celiac, cranial, and caudal mesenteric ganglion). They release acetylcholine as a neurotransmitter that interacts with nicotinic receptors on the postganglionic nerves. Postganglionic fibers innervate some enteric neurons or directly innervate effector cells in the GI tract, such as vascular smooth muscle cells. Norepinephrine is the major postganglionic neurotransmitter. Sympathetic innervation is often inhibitory to GI functions.
Regarding extrinsic sensory nerves, parasympathetic and sympathetic nerve tracts also carry sensory fibers from the gut to cell bodies that are located in nodose ganglia and the dorsal root ganglia, respectively. Cell bodies in the nodose and dorsal root ganglia then project fibers to the brain stem (from nodose ganglia) or spinal cord (from dorsal route ganglia). Sensory nerve fibers in the wall of the GI tract detect mucosal pH and osmolality and can respond to amino acids or glucose, temperature, tension, and touch. In this manner, the extrinsic sensory nerves sense changes in the environment of the intestine and trigger central reflexes that initiate secretomotor changes to maintain normal homeostasis. Extrinsic sensory nerves also contribute to GI inflammation and pain. Sensory nerve endings in the wall of the gut detect noxious chemical and mechanical stimuli, including acid, inflammatory agents, and distension. These stimuli trigger the release of the neuropeptides, substance P, and calcitonin gene-related peptide, from the endings of sensory nerves within the gut wall, where they induce extravasation of plasma proteins and infiltration of granulocytes and arteriolar vasodilatation to cause neurogenic inflammation. The same stimuli induce release of neuropeptides from the central projections of these neurons, where they participate in pain transmission. Additional research is required to define the mechanisms of neurogenic inflammation and GI pain.
Hormones are blood-borne messengers released from endocrine cells or glands into the circulation, which carries them to distant target cells (Figure 13–5). This mechanism of endocrine regulation was discovered in the GI tract in 1902, when Bayliss and Starling discovered the hormone secretin in the small intestine and showed that it stimulates secretion from the exocrine pancreas. Since then, a large number of hormones have been identified in all regions of the GI tract. In this respect, the GI tract is the largest endocrine organ.
GI hormones have several characteristics in common. They are secreted from endocrine cells that are scattered throughout the mucosa of the stomach and intestine rather than being concentrated in specialized glands. This diffuse distribution made purification a truly Herculean task: Many hundreds of kilograms of intestine were required to isolate a few milligrams of pure hormone. GI hormones are invariably peptides, and many of these peptides are present not only in endocrine cells but also in nerves of the enteric system and CNS (Table 13–3). Thus, they have dual functions as hormones and neurotransmitters. After feeding, there are elevated levels of many GI hormones in the circulation. When administered to reproduce postprandial plasma concentrations, these hormones have multiple biological effects, ranging from the stimulation of gastric acid secretion to the suppression of appetite. The physiologic role of some GI hormones has been clearly established by demonstration that antagonists of hormone receptors block certain physiologic processes. However, in many cases, such antagonists are not available, and the physiologic relevance of hormones that cannot be antagonized remains to be determined.
Products | Physiologic Actions | Site of Release | Stimulus for Release | Disease Association |
---|---|---|---|---|
True hormones | ||||
Gastrin | Stimulates acid secretion and growth of gastric oxyntic gland mucosa | Gastric antrum (and duodenum) | Peptides, amino acids, distension, vagal stimulation | Zollinger-Ellison syndrome, peptic ulcer disease |
CCK | Stimulates gallbladder contraction, pancreatic enzyme and bicarbonate secretion, and growth of exocrine pancreas | Duodenum and jejunum | Peptides, amino acids, long-chain fatty acids, (acid) | |
Secretin | Stimulates pancreatic bicarbonate secretion, biliary bicarbonate secretion, growth of exocrine pancreas, pepsin secretion; inhibits gastric acid secretion, trophic effects of gastrin | Duodenum | Acid (fat) | |
GIP | Stimulates insulin release; (inhibits gastric acid secretion) | Duodenum and jejunum | Glucose, amino acids, fatty acids | |
Candidate hormones | ||||
Motilin | Stimulates gastric and duodenal motility | Duodenum and jejunum | Unknown | Irritable bowel syndrome; diabetic gastroparesis |
Pancreatic polypeptide | Inhibits pancreatic bicarbonate and enzyme secretion | Pancreatic islets of Langerhans | Protein (fat and glucose) | |
Enteroglucagon | Elevates blood glucose? | Ileum | Glucose and fat | |
Paracrines | ||||
Somatostatin | Inhibits release of most other peptide hormones | GI tract mucosa, pancreatic islets of Langerhans | Acid stimulates, vagus inhibits release | Gallstones |
Prostaglandins | Promote blood flow, increase mucus and bicarbonate secretion from gastric mucosa | Multiple | Various | NSAID-induced gastritis and ulcer disease |
Histamine | Stimulates gastric acid secretion | Oxyntic gland mucosa | Gastrin and unknown others | |
Neurocrines | ||||
VIP | Relaxes sphincters and gut circular muscle; stimulates intestinal and pancreatic secretion | Mucosa and smooth muscle of GI tract | Enteric nervous system | Secretory diarrhea |
Bombesin | Stimulates gastrin release | Gastric mucosa | Enteric nervous system | |
Enkephalins | Stimulate smooth muscle contraction; inhibit intestinal secretion | Mucosa and smooth muscle of GI tract | Enteric nervous system | |
Other products | ||||
Intrinsic factor | Binds vitamin B12 to facilitate its absorption | Parietal cells of the stomach | Constitutive secretion | Autoimmune destruction resulting in pernicious anemia |
Mucin | Lubrication and protection | Goblet cells along entire intestinal mucosa and surface cells in stomach | GI tract irritation | Viscid mucus in cystic fibrosis. Attenuation in some cases of peptic ulcer |
Acid | Prevents infection; initiates digestion | Parietal cells of the stomach | Gastrin, histamine, acetylcholine, NSAIDs (indirectly) | Acid-peptic disease |
Many substances that are used for intercellular signaling are rapidly removed from the extracellular fluid by uptake into nearby cells or by enzymatic degradation. Such substances have a short half-life in the extracellular fluid and are consequently only capable of regulating neighboring cells. Paracrine substances are released from nonneuronal sensory cells and neurons and regulate the function of neighboring cells rather than influencing distant organs by passage through the circulation (Figure 13–5 and Table 13–3). Examples include histamine and somatostatin, which are released from cells in the stomach to control acid secretion, and serotonin (5-hydroxytryptamine [5-HT]), which is released in the small intestine to control activity of the vagus nerve.
Checkpoint
9. What are the three general mechanisms of control observed in the GI tract?
10. What are the two components of the enteric nervous system?
11. What are the three general types of enteric neuron?
12. Describe the parasympathetic and sympathetic innervation of the GI tract.
13. What is the relationship between the enteric and central nervous systems?
The two principal muscle layers that control motility of the GI tract are the inner circular layer and the outer longitudinal layer of the muscularis externa. They vary in thickness in different regions of the GI tract. For example, the muscles are thickened in the gastric antrum, where strong contractions break up food before it can enter the small intestine, and muscle layers are thickened to form sphincters. Most of the GI muscle is smooth muscle, except the pharynx, parts of the esophagus, and the external anal sphincter, which are made up of striated (skeletal) muscle. GI smooth muscle is similar to smooth muscle in other organs: Fusiform cells are packed together in bundles by connective tissue sheaths. Gap junctions between cells allow signals to readily pass from cell to cell so that the contraction of bundles occurs synchronously. Interstitial cells of Cajal form an extensive network of stellate cells in the muscle layers of the stomach and intestine that are intimately associated with smooth muscle cells and enteric neurons (Figure 13–8). They may have two functions. First, they transmit information from enteric neurons to the smooth muscle cells. Second, they are the pacemaker cells, which have the capacity to generate the basic electrical rhythm or slow waves that are a consistent feature of GI smooth muscle. Animals lacking interstitial cells of Cajal show markedly abnormal GI motility, including defective gastric emptying and intestinal stasis or ileus. Defects in interstitial cells of Cajal may be associated with motility disturbances in patients, and this is an area of active investigation.
GI smooth muscle cells have a resting membrane potential of –40 to –80 mV as a result of the relative conductances of K+, Na+, and Cl– ions. An electrogenic Na+-K+ ATPase contributes significantly to the resting membrane potential. Less is known about the electrophysiological properties of interstitial cells of Cajal, in part because of difficulties in isolating these cells for study. The resting membrane potential of smooth muscle cells varies characteristically with time and is called a slow wave or basic electrical rhythm. Slow waves occur at 3–5/min in the stomach and at 12–20/min in the intestine. Interstitial cells of Cajal set the frequency of the slow waves, and slow waves are transmitted between cells through gap junctions. Nerves and hormones modulate the amplitude of slow waves. Depending on the amplitude of the slow waves and the excitability of the smooth muscle, slow waves can give rise to action potentials. If the slow-wave depolarization reaches a threshold, a train of action potentials will fire. Action potentials depolarize the membrane of the smooth muscle cells and induce an influx of Ca2+ ions into the cytoplasm through voltage-sensitive Ca2+ channels in the plasma membrane and from intracellular stores, causing contraction. What causes an action potential to occur? The presence of neurotransmitters or hormones that are released close to the smooth muscle cells alters the resting membrane potentials of the cells, which makes the oscillations in membrane potential (the slow waves) more or less likely to reach threshold and initiate an action potential. However, because inhibitory motor neurons of the GI tract are highly active and can prevent generation of action potentials, not all slow waves result in active contractions. Action potentials and contractions can only occur when these inhibitory motor neurons are switched off by input from interneurons. Thus, the tonic inhibition serves to contrail the inherent excitability of the pacemaker cells.
Several characteristic patterns of contraction can be observed in GI smooth muscle. Tonic contractions are best represented by sphincters that act as one-way valves to prevent retrograde movement of material from distal to more proximal regions and thus to facilitate flow in an aboral direction. The proximal parts of the stomach and the gallbladder also exhibit tonic contractions. Peristaltic contractions are moving waves of contraction that propel digesta along the GI tract. Peristalsis involves neurally mediated contraction of smooth muscle on the oral side of a bolus of digesta and a neurally mediated relaxation of muscle on the anal side of the digesta. Peristalsis occurs in the pharynx, esophagus, gastric antrum, and small and large intestine. Segmental contractions produce narrow contracted segments between relaxed segments. These movements allow mixing of the luminal contents with GI tract secretions and increase exposure to mucosal surfaces where absorption occurs. Segmentation occurs in the stomach and intestine. Pathologic patterns of motility include spasms, which are very strong and often painful contractions that occur continuously in a dysregulated manner, and ileus, where there is a markedly decreased or absent contractile activity. Ileus often results from irritation of the peritoneum involved in surgery, peritonitis, and pancreatitis. Further research is required to understand the mechanisms of these abnormal contractions, which may lead to improved therapies.
Checkpoint
14. What are the positive and negative regulators of smooth muscle cell action potentials?
15. What are the functions of interstitial cells of Cajal?
16. What are the general types of contractions observed in the GI tract after feeding?
The oropharynx provides entry to the GI tract during swallowing and to the respiratory tract during breathing. It includes the vocal cords, which separate the two tracts and provide the structural basis for speech. Much of the oropharynx is lined with a respiratory-type ciliated pseudocolumnar epithelium.
The esophagus is a hollow tube (25–30 cm long, 2–3 cm wide). The wall of the esophagus consists of a stratified squamous epithelial cell layer, an inner layer of circular muscle, a myenteric nerve plexus, and an outer layer of longitudinal muscle. The first third of esophagus is composed of striated muscle, the middle third is mixed striated and smooth muscle, and the lower third is purely smooth muscle. The esophagus is delimited by an upper esophageal sphincter (a distinct thickening of striated circular muscle) and a lower esophageal sphincter (a tonically contracted 3–4 cm ring of smooth muscle). The two sphincters generate small luminal zones of high pressure, whereas the rest of the esophageal lumen is at a pressure equal to the surrounding body cavities. Between swallows, the two sphincters are closed, preventing entry of air and gastric acid into the esophagus. Regulation of the lower esophageal sphincter is especially important because it controls the passage of digesta into the stomach and prevents the reflux of gastric contents into the esophagus, where they can damage the mucosa. Between swallows, the lower esophageal sphincter is contracted, in large part by vagal cholinergic mechanisms. During swallowing, vagal inhibitory fibers allow the lower esophageal sphincter to relax, possibly because of release of inhibitory neurotransmitters from enteric nerves, including nitric oxide and vasoactive intestinal peptide (VIP).
Swallowing begins as a voluntary process that rapidly becomes an involuntary reflex mechanism. During the voluntary oral phase, the tongue pushes a bolus of food to the back of the mouth and into the oropharynx. From there on, the process is involuntary. In the pharyngeal phase, the food bolus stimulates touch receptors in the pharynx. Sensory signals pass by the glossopharyngeal, vagal, and trigeminal nerves to the swallowing center in the medulla and pons. Motor impulses pass through cranial nerves to control an involuntary process that directs food into the esophagus and away from the airway. Breathing is interrupted and the soft palate is elevated, closing the pharyngeal opening of the nasopharynx and preventing food from entering the internal openings of the nostrils. The tongue is pressed against the hard palate, closing the oral opening of the pharynx. The glottis is pulled under the epiglottis, which blocks the laryngeal opening. Cartilages around the larynx are pulled together, further restricting food from entering the respiratory tract. When all openings to the pharynx are closed, a wave of muscular contraction pushes the bolus of food toward the opening of the esophagus. As the food reaches the esophagus, the upper esophageal sphincter relaxes to accept the material and then closes after the bolus has moved through. The esophageal phase of swallowing begins when the bolus passes through the upper esophageal sphincter. Vagal stretch receptors in the wall of the esophagus detect distension by the bolus and induce a vagovagal reflex, during which vagal motor nerves induce a wave of contraction that spreads along the esophagus at 3–5 cm/s. This is termed primary peristalsis (Figure 13–9). As the wave of primary peristalsis reaches the lower esophageal sphincter, the sphincter relaxes to allow the bolus to enter the stomach. Distension of the esophagus by the bolus can initiate another wave of contraction called secondary peristalsis. Often repetitive waves of secondary peristalsis are required to clear the esophagus of food. Various hormones and neurotransmitters, foods, and drugs can affect the tone of the lower esophageal sphincter pressure.
Figure 13–9
Primary peristalsis of the esophagus. The tracings show pressures in the indicated regions of the esophagus at rest and at various times after swallowing. UES, upper esophageal sphincter; LES, lower esophageal sphincter. (Redrawn from data in Conklin JL et al. Motor functions of the pharynx and esophagus. In: Johnson LR, ed. Physiology of the Gastrointestinal Tract, 3rd ed. Lippincott-Raven, 1994.)
The importance of oropharyngeal motility and its control is seen in patients who have had strokes or are demented. Inability to swallow properly often makes them unable to manage their own oral secretions, resulting in aspiration of oral contents into the lungs with development of pneumonia. This is a common cause of death in individuals with these kinds of CNS disorders. Disordered lower esophageal sphincter tone can cause gastroesophageal reflux disease (GERD), presenting as heartburn and potentially increasing risk for adenocarcinoma of the esophagus.
Checkpoint
17. What is the histologic difference between the proximal one-third and the distal two-thirds of the esophagus?
18. What are the functions of the upper and lower esophageal sphincters, and how are they regulated?
19. Describe the three phases of the swallowing reflex.
The stomach is a complex glandular organ that is guarded by two sphincters: the lower esophageal sphincter and the pyloric sphincter (Figure 13–10). The mucosa is formed by a single layer of epithelial cells that line the lumen of the stomach and descend into funnel-shaped invaginations that are broad at the top near the surface and then narrow as they descend deeper. The broad upper portion is called the pit zone. The middle portion, where the invaginations narrow into glands, is the neck, and the deepest zone is the base. The stomach can be divided anatomically into several regions on the basis of structure and function. The cardia is a small region just distal to the lower esophageal sphincter where the gastric glands are almost entirely composed of mucus-secreting cells. The corpus, or body, is the major part of the stomach and includes the fundus, which is the portion of the body superior to the insertion of the esophagus. Gastric glands in the corpus contain parietal cells (mostly in the neck zone), which secrete hydrochloric acid and intrinsic factor, and chief cells (mostly in the base zone), which secrete pepsinogen. The corpus is the principal site of gastric digestion. The pyloric antrum is the distal region of the stomach that secretes the hormone gastrin from G cells. Glands of the antrum, like those of the cardia, secrete mostly mucus. The antrum, a highly muscular portion of the stomach, grinds food and regulates gastric emptying.
A number of products are secreted from the stomach. Of these, hydrochloric acid is perhaps the most important from a pathophysiologic standpoint. Secretion of acid by the parietal cells of the gastric glands occurs in a basal diurnal pattern but can be stimulated by such diverse factors as the thought of food, distension of the stomach, and protein ingestion.
The mechanisms by which parietal cells secrete HCl into the stomach have been intensively studied because of the importance of acid secretion to digestion and in disease states. Parietal cells are roughly pyramidal in shape. Their membranes express a H+-K+ ATPase, a primary active transporter that is responsible for the secretion of HCl. Parietal cells undergo a remarkable change in appearance when stimulated to secrete HCl (Figure 13–11). In the unstimulated state, they harbor an intracellular tubulovesicular network studded with H+-K+ ATPase molecules. On activation, the tubulovesicular membranes fuse with the plasma membrane to form a canalicular membrane with microvilli. The result is an increase in the area of the apical membrane by 50- to 100-fold that allows markedly increased secretion of HCl by the H+-K+ ATPase pumps directly into the glandular lumen, which in turn squirts the acid into the lumen of the stomach.
Figure 13–11
Acid secretion by parietal cells. Top: Upon stimulation, tubulovesicular network in the parietal cell fuses to form an extensive canalicular membrane with microvilli, which increases the surface area. Bottom: The mechanisms of HCl secretion by parietal cells, stimulated by histamine, acetylcholine, and gastrin, are demonstrated. For abbreviations, see legend for Figure 13–12.
The H+-K+ ATPase is a heterodimer of an α-subunit (the catalytically active unit) and a β-subunit (involved in fixing the intracellular location). The H+-K+ ATPase pumps H+ ions from the cell across the apical membrane in exchange for K+ ions (Figure 13–11). This is an example of primary active transport that is driven by ATP, which pumps H+ ions against an enormous concentration gradient (1 million:1). Tight junctions between cells prevent the reentry of H+ ions into the mucosa. The K+ ions that have entered the cells then recycle to the lumen or enter interstitial fluid by K+ channels. To maintain electroneutrality, Cl– ions are secreted passively across the apical membrane into the lumen through Cl– channels, forming HCl. The secreted H+ ions are provided by H2O and CO2, which form H2CO3. Carbonic anhydrase generates H+ ions for secretion and HCO3– ions, which enter the interstitial fluid by exchange for Cl– ions. Cl– ions enter against their electrochemical gradient, driven by efflux of HCO3– down an electrochemical gradient. The secretion of HCO3– into the blood forms the “alkaline tide,” which can lead to alkalosis when H+ ion secretion is excessive. Water movement maintains the osmotic balance in all regions.
An understanding of the mechanisms of HCl secretion by parietal cells permitted the development of proton pump inhibitors (PPIs), a class of drugs that inhibit the H+-K+ ATPase. Drugs such as omeprazole, a benzimidazole, are inactive at neutral pH levels but, when acidified (in the stomach), bind to sulfhydryl groups of cysteine residues on the external surface of the H+-K+ ATPase, irreversibly inhibiting activity and blocking hypersecretion of gastric acid. Other experimental drugs, termed acid pump antagonists, competitively interfere with K+ ion binding to block acid secretion. These drugs can be used to inhibit the hypersecretion of gastric acid, which causes GERD.
The three main stimulants of H+ ion secretion are acetylcholine, gastrin, and histamine, all of which stimulate HCl secretion and induce characteristic shape changes of the stimulated parietal cell. Acetylcholine is released from vagal postganglionic or enteric neurons during feeding. It binds to muscarinic M3-type muscarinic receptors on parietal cells to stimulate H+ ion secretion. Gastrin is a peptide hormone of 17 or 34 amino acids that is secreted from G cells in the gastric antrum during feeding. Gastrin binds to cholecystokinin (CCK) type B receptors on parietal cells, which also stimulates H+ ion secretion.
Both acetylcholine and gastrin receptors activate the same signal transduction pathways: activation of phospholipase Cβ, leading to generation of inositol trisphosphate that mobilizes Ca2+ from intracellular stores, and diacylglycerol, which activates protein kinase C. Because both acetylcholine and gastrin act through similar intracellular pathways, the combined effects of gastrin and acetylcholine are additive.
Histamine is a paracrine substance secreted by enterochromaffin-like (ECL) and mast cells in the corpus mucosa during feeding. Histamine binds to H2 receptors on parietal cells to activate adenylyl cyclase and increase cAMP. The cAMP activates protein kinase A to stimulate H+ ion secretion. The combination of histamine and acetylcholine or gastrin can increase the rate of acid production by up to 10-fold over basal levels, a much greater effect than simple addition of the effects of the agonists would predict. This effect is known as potentiation. Potentiation requires that two different signal molecules bind to receptors that act through different intracellular mechanisms. Increased intracellular Ca2+ and cAMP activate K+ channels on the apical membrane of parietal cells, thereby promoting K+ ion efflux from the cell. This hyperpolarizes the cell (more negative inside) to promote Cl– ion secretion across the apical membrane. Ca2+ and cAMP also increase insertion of Cl– channels and H+-K+ ATPase into the apical membrane. The combined effects are to stimulate HCl secretion.
Gastrin also regulates growth of the gastric epithelium. Excess gastrin produced by certain tumors causes hyperproliferation of gastric glands and parietal cells and excess secretion of gastric acid. The excess acid in the small intestine can lead to ulceration of the mucosa, steatorrhea as a result of inactivation of pancreatic lipases (which are inhibited by low pH), and diarrhea. This condition is termed Zollinger-Ellison syndrome. Excessive administration of PPIs can result in prolonged high luminal pH, which stimulates hypersecretion of gastrin and increased mucosal growth. Termination of drug treatment then results in an acid production rebound because of the increased content of parietal cells and gastrin-secreting G cells.
In addition to the direct mechanisms by which acetylcholine, gastrin, and histamine stimulate HCl secretion from parietal cells, acetylcholine and gastrin also indirectly stimulate secretion by acting on enterochromaffin-like cells to promote the release of histamine, which in turn stimulates parietal cells. The importance of histamine to H+ ion secretion is illustrated by studies with histamine H2 receptor antagonists, such as cimetidine. These drugs not only inhibit histamine-stimulated H+ ion secretion but also block the effects of acetylcholine and gastrin. By preventing such potentiation, these agents can be used to effectively treat the hypersecretion of gastric acid.
Somatostatin, a peptide of 14 or 28 amino acids, is an important inhibitor of gastric acid secretion. Somatostatin directly inhibits proton secretion by activating receptors on parietal cells, which couple to produce inhibition of cAMP. Somatostatin also inhibits gastrin and histamine secretion, which indirectly inhibits proton secretion. Somatostatin is secreted by D cells in the gastric antrum and corpus. D cells in the gastric antrum have direct contact with the stomach lumen (open endocrine cells), allowing them to sense the luminal contents. Protons in the antrum stimulate somatostatin secretion, which acts as a paracrine agent to inhibit gastrin secretion from neighboring G cells and to thereby indirectly reduce gastric acid secretion. This is an example of negative-feedback regulation. D cells in the corpus do not have contact with the lumen (closed cells) and thus cannot sense luminal protons. Instead, multiple neurohumoral factors (eg, noradrenalin, CCK, VIP) increase release of corpus somatostatin, which in turn inhibits acid production indirectly by decreasing histamine release from ECL cells and directly by inhibiting parietal cells. Vagal ACh and the TH1 cytokine interferon-γ inhibit somatostatin release and promote acid secretion. Recent studies indicate that the endocrine cells in the stomach sense luminal nutrient and acid via primary cilia on their apical surfaces.
Secretion of gastric acid between meals is low. Three phases of acid secretion occur during feeding (Figure 13–12). The cephalic phase (~30% of response) of secretion is initiated by the sight, smell, taste, and swallowing of food. These stimuli activate the dorsal motor nucleus of the vagal nerve in the medulla and result in vagal discharge and parasympathetic motor nerves. Stimulation has several consequences. In the corpus, postganglionic nerves release acetylcholine, which directly activates parietal cells by M3 receptors. Acetylcholine also induces histamine release from enterochromaffin cells, which stimulates H+ ion secretion by parietal cells. In the antrum, vagal stimulation induces release of the peptide, gastrin-releasing peptide, from postganglionic fibers, which stimulates gastrin release and thus indirectly stimulates secretion of H+ ion secretion. Acetylcholine also inhibits somatostatin release from D cells in the corpus and pylorus to stimulate secretion of H+ ions.
Figure 13–12
Regulation of gastric acid secretion by nerves and hormones. During the cephalic phase of digestion, vagal cholinergic nerves directly stimulate parietal cells and induce release of histamine from ECL cells, which also stimulate parietal cells. Vagal fibers also release gastrin-releasing peptide (GRP) in the antrum to induce gastrin secretion, which is carried in the bloodstream to induce release of histamine and stimulate parietal cells. During the gastric phase of digestion, food in the stomach triggers vagovagal reflexes and also stimulates gastrin secretion. Acidification of the gastric antrum stimulates the release of somatostatin, which inhibits gastrin release and thus acid secretion; vagal ACh inhibits somatostatin release. (ACh, acetylcholine; G, gastrin; S, somatostatin; M3-R, muscarinic 3 receptor; H2-R, histamine 2 receptor; CCKB-R, cholecystokinin B receptor; ECL, enterochromaffin-like; GRP-R, GRP receptor; GRP, gastrin-releasing peptide.)
The gastric phase (~70% of response) of secretion is induced by stimuli within the stomach. Vagal sensory nerves detect gastric distension with food and trigger a vagovagal reflex during which vagal motor nerves release acetylcholine in the stomach to promote acid secretion. Partially digested proteins and amino acids stimulate gastrin release from G cells in the pylorus. G cells, like D cells, are open-type endocrine cells that directly sense the contents of the stomach. Gastrin then further stimulates acid secretion. Acidification of the pylorus stimulates somatostatin release, which inhibits acid secretion by a negative-feedback loop as described.
During the intestinal phase, the products of protein digestion, on entering the small intestine, can stimulate gastrin release from G cells in the duodenum. Many substances, most notably fat and acid, stimulate the secretion of hormones from the small intestine that inhibit gastric acid secretion. Examples include secretin and cholecystokinin.
Helicobacter pylori is a bacterium that lives in the mucous layer of the stomach where the enzyme urease is active, converting urea to CO2 and ammonia. Ammonia buffers luminal acid and protects the organism. H pylori also secretes proteins, such as CagA and VacA, that modulate immune responses and directly alter mucosal cell signaling pathways. More than half of the world’s population is infected with H pylori. In most cases, the infection, though chronic, is mild and does not cause symptoms. In some individuals, however, the infection leads to increased acid secretion and symptomatic inflammation that causes ulceration of the stomach or duodenum. Almost all duodenal peptic (i.e., acid-associated) ulcers and about half of gastric peptic ulcers have H pylori infection as a root cause; the remaining gastric ulcers are caused by medications (such as aspirin and nonsteroidal anti-inflammatory drugs). In some patients, chronic H pylori infection can lead to death (atrophy) of parietal cells, chronic inflammation, and altered mucosal differentiation patterns (metaplasias) that increase the risk of progression to gastric cancer. In certain geographical regions (eg, East Asia and parts of Central and South America), due to environmental and/or lifestyle factors that have not yet been elucidated, risk for progression to gastric cancer is much higher than in other regions (eg, United States and Canada).
Chief cells in the glands of the gastric corpus secrete pepsinogen, an inactive precursor (zymogen) of the active protease, pepsin. Acetylcholine is the main stimulant of pepsinogen secretion, although other factors (eg, gastrin) also stimulate secretion. Once released into the lumen of the stomach, gastric acid and preexisting pepsin convert pepsinogen to pepsin. Pepsin has a pH optimum of 3.0 and is thus active in the stomach. It is an endopeptidase that begins the degradation of dietary proteins to peptides. However, pepsin accounts for only 10% of the total protein digestion.
Mucins are high-molecular-weight glycoproteins secreted by mucous cells of gastric glands in the corpus and antrum. The peptide backbone of mucins is densely populated with carbohydrate side chains enriched with sulfate groups. Mucins combine with phospholipids, bicarbonate, and water to form the mucus gel layer that adheres to the surface of epithelial cells of the stomach. This layer forms physical protection for epithelial cells from damage by contractile grinding of food as well as noxious substances such as acid, pepsin, and bile acids. Acetylcholine and mucosal irritation stimulate secretion of mucin.
Epithelial cells of the corpus and antrum secrete HCO3– ions. Although the secretion of HCO3– is minor compared with H+ ion secretion, HCO3– plays a major role in epithelial defense. HCO3– ions are trapped in the mucous gel to form an “unstirred layer” in proximity to the epithelium, where the pH is 7.0 compared with 1.0–3.0 in the lumen. Acetylcholine and intraluminal acid stimulate HCO3– secretion.
Intrinsic factor is a glycoprotein secreted by parietal cells that is required for vitamin B12 absorption. Vitamin B12 (cobalamin) is not made in mammalian cells, and the only source is the diet: meat, fish, dairy products, but not vegetables or fruit. In the stomach, acid and pepsin release B12 from dietary carrier proteins. The acid environment permits binding of B12 to haptocorrin (R factor), a glycoprotein produced by salivary glands and gastric glands. The B12-haptocorrin complex enters the duodenum, where pancreatic proteases digest the haptocorrin. Free intrinsic factor also enters the duodenum. Intrinsic factor combines with B12 in the less acidic environment of the small intestine, forming a degradation-resistant complex for transport to the ileum. Specific receptors on epithelial cells lining the ileum bind the vitamin B12–intrinsic factor complex, which is taken into cells by endocytosis. The absorbed complex dissociates within the epithelial cells, and then vitamin B12 binds to transcobalamin II, a protein required for exocytosis and transport to the liver. In autoimmune gastritis, parietal cells are destroyed, leading to loss of intrinsic factor secretion, which can result in vitamin B12 deficiency and pernicious anemia. This anemia is caused by impaired synthesis of purines and thymine for which vitamin B12 is required. The only reliable therapy is regular intramuscular injections of vitamin B12.
In terms of motility, the proximal and distal regions of the stomach are distinct. The gastric corpus is a reservoir for gastric digestion. During each swallow, stretch of the esophagus induces a vagovagal reflex that causes the gastric corpus to relax in preparation to receive the food, a phenomenon known as receptive relaxation. When food enters the stomach, it relaxes further to accommodate a meal of 1.5 L without any increase in pressure, a phenomenon called accommodation, which involves vagovagal and local enteric reflexes. Thus, the stomach is a reservoir for ingested food. The antrum of the stomach is highly muscular, and here contractions serve to break food to smaller pieces and thereby facilitate digestion. The pyloric sphincter controls the rate at which the antral contractions propel partially digested food, or chyme, into the duodenum. During fasting, the antrum is relatively quiescent, with occasional forceful contractions that occur every 75–90 min. These intense contractions, of 5- to 10-min duration, are part of a general wave of contractions that sweep the entire length of the GI tract during fasting: the migrating myoelectric complex. Feeding disrupts the migrating myoelectric complex, and now the antrum contracts frequently at a rate of about three contractions per minute. These slow waves of peristaltic contraction originate from spontaneously active interstitial cells of Cajal in the pacemaker zone in the middle of the body of the stomach, and they sweep toward the antrum. When the membrane potential of muscle cells depolarizes to reach threshold, action potentials fire. Contractions occur during the plateau phase of the action potential. Gastrin and acetylcholine stimulate contraction by increasing the magnitude and duration of the action potentials.
Immediately after a meal, the stomach may contain up to 1 L of material, which empties slowly into the small intestine. Regulation of gastric emptying occurs by alterations in motility of the proximal and distal stomach, pylorus, and duodenum. Gastric emptying is brought about by an increase in tone (intraluminal pressure) in the proximal stomach, increase in strength of antral contractions, opening of the pylorus, and inhibition of duodenal segmental contractions.
The rate of gastric emptying depends on the chemical and physical composition of chyme that enters the duodenum through the stimulation of both neural and hormonal pathways. Solids and liquids empty at different rates: Liquids empty rapidly, and solids empty only after a lag phase. Acid, fat, and hyperosmolar solutions entering the duodenum slow gastric emptying through stimulation of neuronal and hormonal mechanisms. Sensory neurons in the duodenum, both vagal and spinal, respond to nutrients, H+ ions, and hyperosmolar content of chyme. Vagal motor nerves decrease antral contractions, contract the pylorus, and decrease proximal gastric motility. This results in intestinal feedback inhibition (slowing) of gastric emptying. The main vagal mediator that stimulates contraction is acetylcholine. VIP and nitric oxide are neuronal mediators that inhibit contraction. Many hormones that are released by endocrine cells in the small intestine have been implicated in the feedback inhibition of gastric emptying. Secretin, the release of which is stimulated by acid, inhibits antral contractions and stimulates contraction of the pyloric sphincter to slow emptying. Cholecystokinin, the release of which is stimulated by fat, acts on receptors on vagal sensory nerves to produce a vagovagal reflex that decreases gastric emptying.
The importance of nervous system control over gastric motility is reflected in the high incidence of the dumping syndrome (nausea, bloating, flushing, and explosive diarrhea) that occurs as a consequence of stomach dysmotility in some patients who have undergone surgical procedures such as partial gastrectomy or nonselective vagotomy.
Checkpoint
20. Describe the cell types found in the mucosa of the gastric corpus and antrum, and indicate the products of each cell type.
21. What are the roles of the proximal and distal stomach?
22. Describe the ionic basis of secretion of HCl from the gastric parietal cells.
23. Name a neurotransmitter, hormone, and paracrine agent that stimulates acid secretion from parietal cells.
24. Name a peptide that inhibits acid secretion from the parietal cells.
25. Describe the mechanisms of the cephalic, gastric, and intestinal phases of gastric acid secretion.
26. Name two types of drugs with distinct mechanisms of action that can be used to treat hypersecretion of gastric acid.
27. What is the role of the parietal cell in absorption of vitamin B12?
28. Describe two processes by which the gastric mucosa is protected from acid in the lumen.
29. What are the patterns of motility in the corpus and the antrum?
30. How does the composition of the digesta in the lumen of the small intestine affect the rate of gastric emptying?
The gallbladder is a muscular sac with a resting volume of about 50 mL that lies on the inferior surface of the liver. It is connected to the hepatic biliary system by the cystic duct, which leads to the common bile duct whose opening into the proximal duodenum is controlled by the sphincter of Oddi. The common bile duct and the pancreatic duct usually join just proximal to this sphincter.
Bile, which is produced by the liver, flows down the hepatic duct and into the gallbladder through the cystic duct. It is stored there until stimulation of gallbladder contraction expels the contents of the gallbladder back through the cystic duct into the common bile duct and through the sphincter of Oddi into the duodenum. Stimuli for gallbladder contraction and sphincter of Oddi relaxation necessary for proper bile flow include both hormones and neural inputs. Fat in the intestine stimulates secretion of the hormone CCK from I-cells. CCK causes contraction of the gallbladder and relaxation of the sphincter of Oddi. Depending on how long it remains in the gallbladder, bile becomes concentrated. Bile composition is further modified by mucin production under the control of prostaglandins and by saturation of bile cholesterol controlled in part by estrogens. The most prominent disorders of the gallbladder involve gallstone formation (see later discussion).
Three regions can be distinguished along the approximately 6–7 m length of the small intestine. The pyloric sphincter marks the beginning of the duodenum, which is largely retroperitoneal and fixed in its location and is 20–25 cm in length. Because of this sphincter, stomach contents normally enter the duodenum in small spurts containing tiny suspended particles. In the duodenum, gastric contents are mixed with the secretions of the common bile duct and pancreatic duct. Beyond the duodenum, the small intestine is mobile and suspended in the peritoneal cavity by a mesentery. The proximal two fifths is called the jejunum. The distal three fifths is called the ileum, which ends at the ileocecal valve at the start of the large intestine.
The most striking gross structural features of the small intestine are the numerous villi (projections of the mucosa into the lumen of the intestine that measure approximately 1 mm in height) (Figure 13–13). Each villus contains a single terminal branch of the arterial, venous, and lymphatic trees. Villi increase the absorptive capacity 5-fold and allow efficient transfer to the circulatory system of substances absorbed from the gut lumen by enterocytes (surface epithelial cells). By electron microscopy, each enterocyte contains 3000–5000 microvilli, plasma membrane evaginations on the apical side of the cell that further increase the absorptive surface area by 200-fold. Many digestive enzymes expressed by intestinal epithelial cells are located at the tips of these microvilli. As a group, these densely packed microvilli make up a “brush border” facing the intestinal lumen.
Invaginations of the intestinal epithelium into the wall surround the villi and are called the crypts of Lieberkühn. These structures are the location of epithelial intestinal stem cells and their proliferative daughters that together constantly produce new differentiated epithelial cells that form the epithelial lining of the intestine. Each small intestinal crypt contains tetrapotential stem cells at or near the crypt base that produces the four mature epithelial cell types: absorptive enterocytes, mucus-secreting goblet cells; hormone-secreting enteroendocrine cells, and antimicrobial peptides and growth factor–secreting Paneth cells. Enterocytes, goblet, and enteroendocrine cells migrate out of crypts and onto adjacent villi. These cells then die by apoptosis at the tips of villi and are extruded into the lumen of the intestine; the average life span is about 4–6 days. On the other hand, Paneth cells are much longer lived (~60 days) and they migrate to the crypt base where they are in close contact with epithelial stem cells.
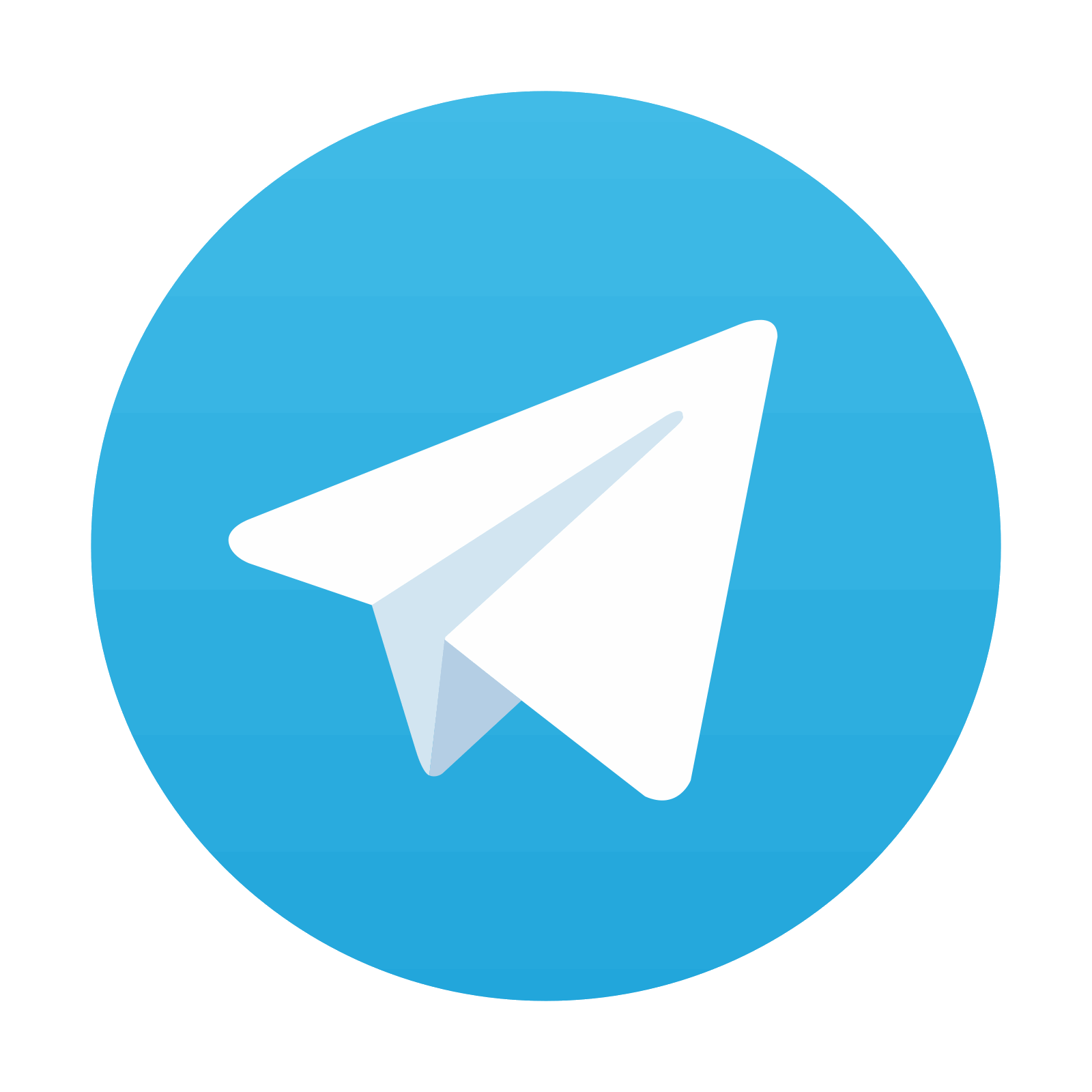
Stay updated, free articles. Join our Telegram channel
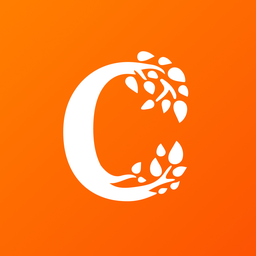
Full access? Get Clinical Tree
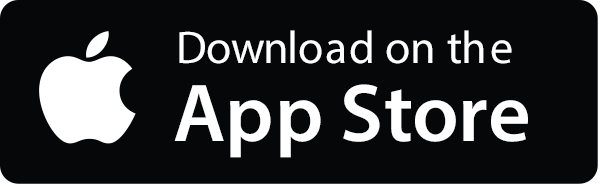
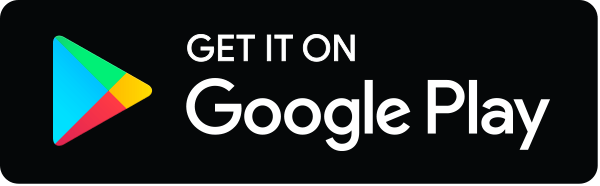