Fig. 2.1
Redox chemistry of free radicals in vivo. Oxygen (O2) reacts slowly to accept a single electron and produce superoxide (O2 •−) in vivo [1], while two electrons can reduce oxygen with the addition of two protons (2H+) to generate hydrogen peroxide (H2O2) [2]. Reactive metal ions (M n+), such as ferric iron and cupric ion, play a major role in oxidative stress via Haber–Weiss reaction, which is reduced by O2 •− to reduced form ion (M(n−1)+) and oxygen [3]. If H2O2 coexists, reduced metal ion will be oxidized to generate a hydroxyl radical (HO•) and a hydroxide anion (HO•) via Fenton chemistry [4]. In addition, superoxide can react with nitric oxide (NO•) to form peroxynitrite (ONOO−), which is a major RNS pathway in vivo and also considered harmful without fulfilling signaling roles
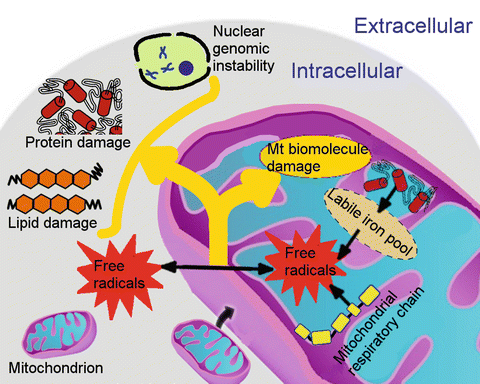
Fig. 2.2
Mitochondrial oxidative stress. The mitochondrial respiratory chain is one major source of free radicals. Mitochondria also express nitric oxide synthase, which produces nitric oxide (NO•). Moreover, mitochondria are the major site of heme and iron–sulfur cluster biosynthesis. Iron taken up by eukaryotic cells is transported into the mitochondrial matrix by iron import proteins (e.g., mitoferrin) for mitochondrial iron storage, iron–sulfur cluster biosynthesis, heme metabolism, or other currently unknown pathways. Defects in these processes (e.g., faulty biosynthesis of heme and iron–sulfur cluster) or impaired mitochondrial iron homeostasis contributes to elevated labile iron levels in mitochondria, which has a strong potential to catalyze the reaction of free radicals. Free radicals within mitochondria are directly associated with extensive damage to mitochondrial DNA, which may further lead to mitochondrial dysfunction. More stable ROSs can reach other intracellular areas, resulting in cytosolic damage of other organelles
Given the fact that reactive intermediates are transient with extremely short half-lives (10−6–10−12 s), it is very difficult to detect them directly in vivo (Leeuwenburgh and Heinecke 2001). A substantial research effort has been directed at exploring end products of oxidative damage to biomolecules, including lipids, proteins, and DNA. The detection of radicals can be performed by direct and indirect measures. Electron spin resonance (ESR) spectroscopy provides a valid tool to investigate free radicals directly by measuring the absorption microwave radiation of unpaired electrons when free radicals are exposed to a strong magnetic field (Davies et al. 1982). Lai et al. successfully used ESR to demonstrate that the superoxide dismutases (SODs) predominantly localized in epithelial cells of rat nasal mucosa scavenge microenvironmental O2 •− radicals (Lai et al. 1997). Moreover, spin trap compounds generally are necessary to detect short-lived radicals. A spin trap reagent can react with the radical to form a spin adduct that is relatively stable and can be detected with ESR spectroscopy. However, in biological systems where multiple radicals or oxidants are present, many may form the same radical adduct with a spin trap reagent. Thus, use of spin trap compounds with ESR spectroscopy may raise an issue of their selectivity and specificity.
Except for biological fluids, radical detection protocols are based mostly on cell-disruptive methods, which inevitably alter the equilibrium between radical species as well as between radicals and various cellular components. Current nondisruptive techniques for detecting radicals and labile ions in vivo dramatically rely on the application of fluorescent probes, which comprise a fluorescent group coupled with a high-affinity chelator (Cabantchik et al. 2002; Kakhlon and Cabantchik 2002). Moreover, probes must be lipophilic, membrane-permeable, and able to compete with endogenous ligands and polypeptides (Petrat et al. 2002; Rauen et al. 2007). For instance, labile iron in cells and tissues can be visualized by fluorescent probes, such as Phen Green SK and calcein. A recent study by Cantu et al. employing an iron indicator rhodamine B 4-[(1,10-phenanthrolin-5-yl)aminocarbonyl]benzyl ester has shown that mitochondrial aconitase releases labile iron under oxidative stress in primary ventral mesencephalic cells (Cantu et al. 2009). This event was followed by mitochondrial dysfunction and cell death. Furthermore, this study’s observation that removal of H2O2 and labile iron, using catalase and an iron chelator (N,N′-bis(2-hydroxybenzyl)ethylenediamine-N,N′-diacetic acid), mitigated cell death strongly suggests that H2O2 and labile iron mediate oxidative damage and cell death.
More indirectly, radical activity—damage—can be detected on a variety of biomolecular fingerprints. The polyunsaturated acids of the fatty acid membrane contain more than one double bond in their alkyl chain, which are extremely vulnerable to ROSs. The end products of oxidant reactions with these vulnerable structures (lipid peroxidation reactions) include alkanes, isoprostanes, malondialdehyde (MDA), and isoketals. The thiobarbituric acid reactive substance (TBARS) assay is a typical nonspecific test that measures lipid peroxidation by detecting MDA levels. Thiobarbituric acid reacts with MDA in acidic condition to form a pink pigment, which has an absorption maximum of 532 nm. Absorbance changes at 532 nm are linearly correlated with chromogen concentration, and the amount of chromogen can be quantified by spectrophotometer (Buege and Aust 1978). In addition, gas chromatography/mass spectrometry (GC/MS) is a powerful technique for measuring MDA or other more robust lipid peroxidation markers, e.g., F2-isoprostane, which present in all normal biological fluids and tissues (Morrow and Roberts 1997).
Reactive radicals’ attacks can modify amino acid residues in the proteins. Reactive hydroxyl radical (HO•) converts the amino acid phenylalanine to ortho-, meta-, and para-tyrosine. Peroxynitrite (ONOO−), which is formed by the reaction of O2 • with NO•, can mediate the nitration of the ortho position of tyrosine residue to form 3-nitrotyrosine. Trace amounts of tyrosine isomers and 3-nitrotyrosine can be detected by GC/MS and high-performance liquid chromatography (HPLC).
Oxidation of specific amino acid residues (lysine, arginine, proline, and threonine) of proteins forms carbonyl species (aldehydes and ketones). Lipid oxidation with the covalent adduction of lipid aldehydes and sugar reduction also contribute to the increase in carbonyl levels (Dalle-Donne et al. 2003). Hence, the carbonyl assay indicates not only protein oxidation but also lipid peroxidation products. Carbonyl levels, however, can be quantified by various techniques, including spectrophotometry, Western blot immunoassay, enzyme-linked immunosorbent assay (ELISA), and GC/MS, which are accessible and can be performed in any biomedical research laboratories. Thus, carbonyl assays are widely accepted for evaluating protein oxidation, with the caveat that additional analysis is required to exclude the carbonyl groups from lipids and sugar.
Free-radical-induced damage to DNA and RNA yields numerous products. The most popular biomarkers of DNA and RNA are guanine base oxidation products, 8-oxo-7,8-dihydro-2′-deoxyguanosine (8-oxodGuo) and 8-oxo-7,8-dihydro-guanosine (8-oxoGuo), respectively, which can be quantified simultaneously using HPLC with an electrochemical detector (Hofer et al. 2006).
2.3 Select Misconceptions in Free-Radical Biology
As briefly discussed earlier, under normal (non-pathophysiological) conditions, a minor amount of free radicals within cells is essential for cellular and intracellular signaling systems. In addition, cells are well protected against oxidative damage by delicate and sophisticated defense systems. The biological activity of free radicals is maintained in balance by endogenous antioxidants and antioxidant enzymes. A sudden acceleration in the production of free radicals (i.e., ischemia reperfusion) or a chronic deficiency in the antioxidant defense system may alter this balance, which leads to free-radical-induced damage to biomolecules, and may be further involved in the pathogenesis of various disorders and diseases (Valko et al. 2007). Levels of beta-carotene, retinol, vitamin E, and vitamin C, for example, were observed to be significantly decreased in children with acute otitis media and tonsillitis (Cemek et al. 2005). Manjunath et al. reported that serum MDA levels were significantly elevated and that ferric reducing antioxidant power was dramatically decreased in patients with laryngeal and hypopharyngeal cancer (Manjunath et al. 2010). Moreover, Aksoy et al. revealed an association of patients with allergic rhinitis to increased serum protein oxidation levels (Aksoy et al. 2009, 2012). Other findings add to the evidence that elevated serum oxidant levels and declined antioxidant status levels were observed in patients with chronic long-term otitis media (Garca et al. 2013a; Baysal et al. 2013; Testa et al. 2012).
Current understanding of the involvement of free radicals in pathology suggests that antioxidant therapy through attenuating free radicals may be important to optimize treatment (Ding et al. 2013; Tian et al. 2013; Someya et al. 2010; Cemek et al. 2005). Most studies using animal models indicate the benefits of antioxidants in the prevention of acute oxidative conditions and diseases. Bonabi et al., for instance, reported that resveratrol had a significant protective effect against gentamicin-induced toxicity in cultured organ of Corti explants from newborn Sprague–Dawley rats (Bonabi et al. 2008). A recent study on noise-induced hearing loss in Fischer 344 rats showed that resveratrol dramatically reduced noise-induced cyclooxygenase-2 expression and ROS formation (Seidman et al. 2013). Moreover, the antioxidant N-acetylcysteine combined with disodium 2,4-disulfophenyl-N-tert-butylnitrone as a therapeutic intervention for noise-induced hearing loss in rats was found to be effective in reducing both temporary and permanent threshold shifts across all frequencies (2–16 kHz) in auditory brainstem response tests (Lu et al. 2014). A clinical study of radix astragali, a natural antioxidant herb, indicated its beneficial effect in recovering hearing in patients with sudden deafness (Xiong et al. 2012). For decades, a substantial research effort has focused on coenzyme Q10 as a therapeutic approach to ENT pathology. A study in a guinea pig model of noise-induced hearing loss by Fetoni et al. demonstrated that coenzyme Q10 decreased active caspase-3 expression and the number of apoptotic cells and improved outer hair cell survival (Fetoni et al. 2009). The same research group also found that coenzyme Q10 significantly mitigated noise-induced auditory function and hearing loss in rats (Fetoni et al. 2012). (Other studies demonstrating the efficacy of antioxidant intervention in the prevention of noise- and drug-induced and age-related hearing loss can be found in Chaps. 10, 11, 12, 13, 14, 15 and 16 of this book.)
Though scientists have made considerable progress in support of antioxidant supplements in pathology, human research findings remain controversial and inconsistent, particularly in epidemiological and clinical studies. A prospective study of vitamin intake and the risk of hearing loss in men aged 40–74 years by Shargorodsky et al. found a lack of correlation between higher intake of vitamins C, E, beta-carotene, or B12 and the risk of hearing loss (Shargorodsky et al. 2010). Consistently, 5-year longitudinal analyses on the link between diet quality and hearing loss in the elderly did not provide robust efficacy data (Gopinath et al. 2014). In a previous study on the glutathione supplement in 30 patients with hearing loss, the treatment failed to modify significantly the progression of hearing loss (Sataloff et al. 2010). On the other hand, studies by Choi et al. and Spankovich et al. both demonstrate relations between use of antioxidant supplements and reduced risk of age-related hearing loss in elderly humans. Despite the differences in the protocols, the conflicting study observations may stem from several factors or misconceptions involved in free-radical biology.
2.4 Antioxidants May Blunt Benefits that Depend on ROS Signaling
Previous research has demonstrated that ROSs actively participate in a variety of biological signaling processes. Appropriate increases in oxidative stress as effector molecules can trigger stress-inducible genes, which participate in cellular repair. Thus, modest stimulation of ROSs is beneficial. Indeed, caloric restriction, with an associate increase in ROS formation, has been shown to extend life span in C. elegans (Schulz et al. 2007). Exposure to H2O2 can also activate transcription of the DNA-damage-response gene and induce DNA repair in HeLa cells (Furukawa-Hibi et al. 2002). Another example of hormetic increases in ROSs is moderate aerobic exercise, which exerts beneficial health effects mediated through direct ROS increases (Mendelsohn and Larrick 2013). In general, acute exercise increases oxidant levels and oxidative stress in untrained animals, but long-term exercise may counter this effect by increasing the activity of antioxidant enzymes and reducing oxidant production. These defenses may be critical for preventing chronic oxidative muscle damage during exercise and even at rest. We have assayed the activities of antioxidant enzymes in rat skeletal muscle samples following exercise training. In most of our studies, the activities of two major antioxidant enzymes, mitochondrial SOD and cytosolic glutathione peroxidase, were significantly higher in exercising animals than in sedentary animals. There was little difference in catalase and cytosolic SOD activities (Leeuwenburgh and Heinecke 2001). This suggests that mitochondria are a source of constant free-radical production, which may cause beneficial adaptations. We also consistently detect lower levels of oxidative stress markers following the exercise training. These results indicate that increased activities of GPX and mitochondrial SOD might account in part for the decline in oxidative damage in exercise-trained animals. What may be surprising is the fact that increased mitochondrial radical production with moderate bouts of exercise can provide long-term beneficial adaptations. During the short burst of exercise, metabolism is characterized by a high rate of adenosine diphosphate (ADP) formation because ATP breakdown increases with workload. High ADP levels activate oxidative phosphorylation, thereby dramatically increasing the levels of electron flow in the respiratory complexes. This flow of electrons toward oxygen reduces the amount of superoxide production but appears sufficient to increase the induction of major antioxidant enzymes GPX and SOD. While some studies now show that antioxidant supplementation can blunt the normally required free-radical production of SOD and GPX induction, more human research is needed. Together these results suggest that antioxidant supplements can blunt the beneficial effects in humans by counteracting with transient hormetic responses in ROSs.
2.5 Many Factors Can Impact the Bioavailability of Antioxidants
In laboratory animal studies, diet compositions are precisely controlled and animals are genetically homogeneous. With heterogeneous backgrounds and different dietary habits in human research participants, individual differences in dietary nutrients, plasma cholesterol profile, gender, and age could have major influences on the bioavailability of antioxidant supplementation.
Antioxidant therapies generally are either hydrophilic or hydrophobic compounds. The use of hydrophobic antioxidants in humans usually proves unsatisfactory, most likely because of their relatively low oral bioavailability and complex absorption process. Coenzyme Q10, for example, is relatively insoluble in aqueous solutions, has a relatively high molecular weight, and has a limited solubility in lipids, which directly results in about 2–3 % absorption when orally administered to rats. Other factors also impact plasma coenzyme Q10 levels after oral administration. There is evidence showing that dietary fat enhances coenzyme Q10 absorption. On the contrary, uptake of vitamin E together with coenzyme Q10 intervened and decreased coenzyme Q10 absorption. These brief examples demonstrate the many effects of administrating a single antioxidant in research studies, thus making interpretation complicated.
2.6 Antioxidants May Act as Prooxidants
Antioxidants are highly reactive and prone to be oxidized. Many factors can influence their stability and trigger oxidation reaction, including light, temperature, pH, and metallic ions.
Previous research in vitro has demonstrated the beneficial outcomes of tea polyphenols, which are potent hydrophilic antioxidants and can scavenge ROSs, RNSs, and redox-active transition metal ions. Epigallocatechin gallate (EGCG) is the primary bioactive compound in green tea, followed by epigallocatechin (EGC), epicatechin gallate (ECG), and epicatechin (EC). Green tea polyphenols are stable in acidic solutions with pH values ranging from 1.0 to 6.0. EGCG with a galloyl moiety is the most vulnerable to pH among them, which results in its instability at physiological pH 7.4. Much attention has therefore focused on the potential prooxidative role of EGCG, particularly in a number of in vitro assays. A decade ago, several lines of evidence suggested EGCG was involved in inducing H2O2 generation and DNA damage in HL-60 cells (Furukawa et al. 2003; Oikawa et al. 2003). Suh et al. reported similar results, in which EGCG was positively associated with apoptotic cell death and H2O2 production in a dose-dependent manner in HIT-T15 pancreatic beta cells (Suh et al. 2010). In the same study, the observation that removing iron using iron chelators inhibited ROS formation strongly suggests that transition metals are essential catalysts during the process. In this case, some oxidants can be used to kill harmful (cancer) cells but may also damage noncancerous cells in the body. Because there is substantial evidence that selected antioxidants also exhibit prooxidant activity, it will be essential to study antioxidant compounds in various cell types and under physiological conditions.
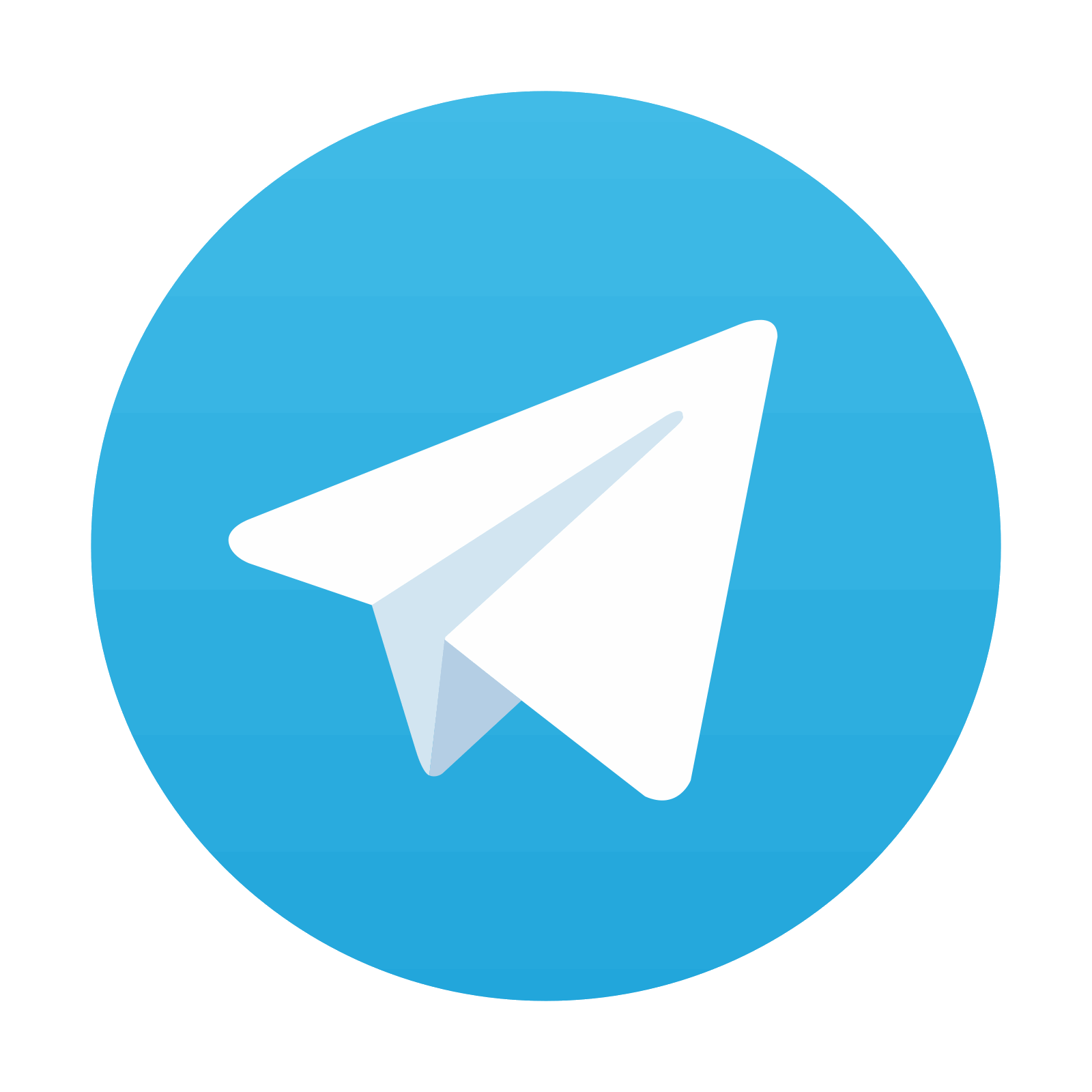
Stay updated, free articles. Join our Telegram channel
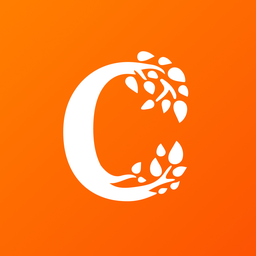
Full access? Get Clinical Tree
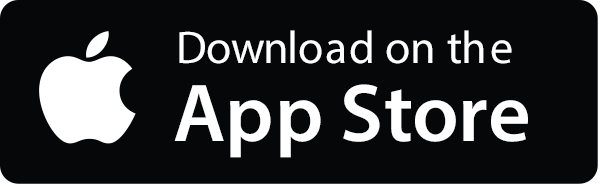
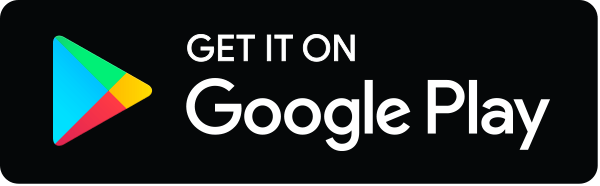