Taking the -log of both sides of the equation yields:
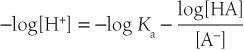
Using the symbol pN for -log10 [N]:
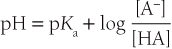
(Note that the unprotonated form is up.)
• Weak acids with low dissociation constants (eg, H2CO3 → HCO3–) with pK of 6.4 act to buffer changes in pH in biological fluids like that outside of cells (pH range 7.35-7.45).
• Amino acids are Zwitterions with dual charges from carboxyl (COO–) and amino (NH3+) groups that react to form peptide bonds (RCO-NHR); peptides (short amino acid chains) and longer polypeptide proteins are also important buffers through their amino acid side groups (Table 1).
TABLE 1. L-α-AMINO ACIDS PRESENT IN HUMAN PROTEINS
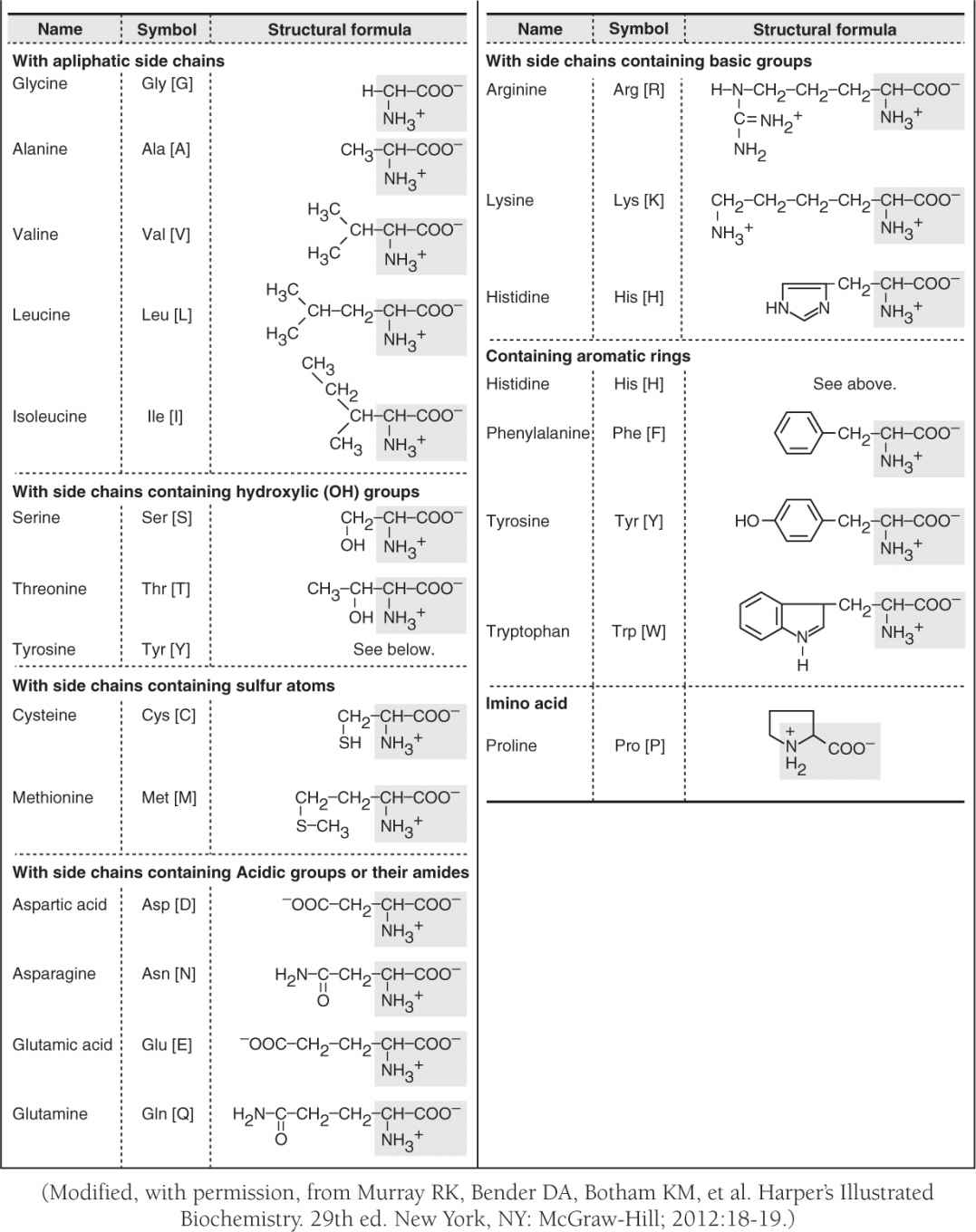
• Interaction of side groups and other factors convert linear amino acid sequences (primary structures) into α-helical or β-sheet segments (secondary structures) to three-dimensional conformations (tertiary structures) with functional regions (domains—see Fig. 1).
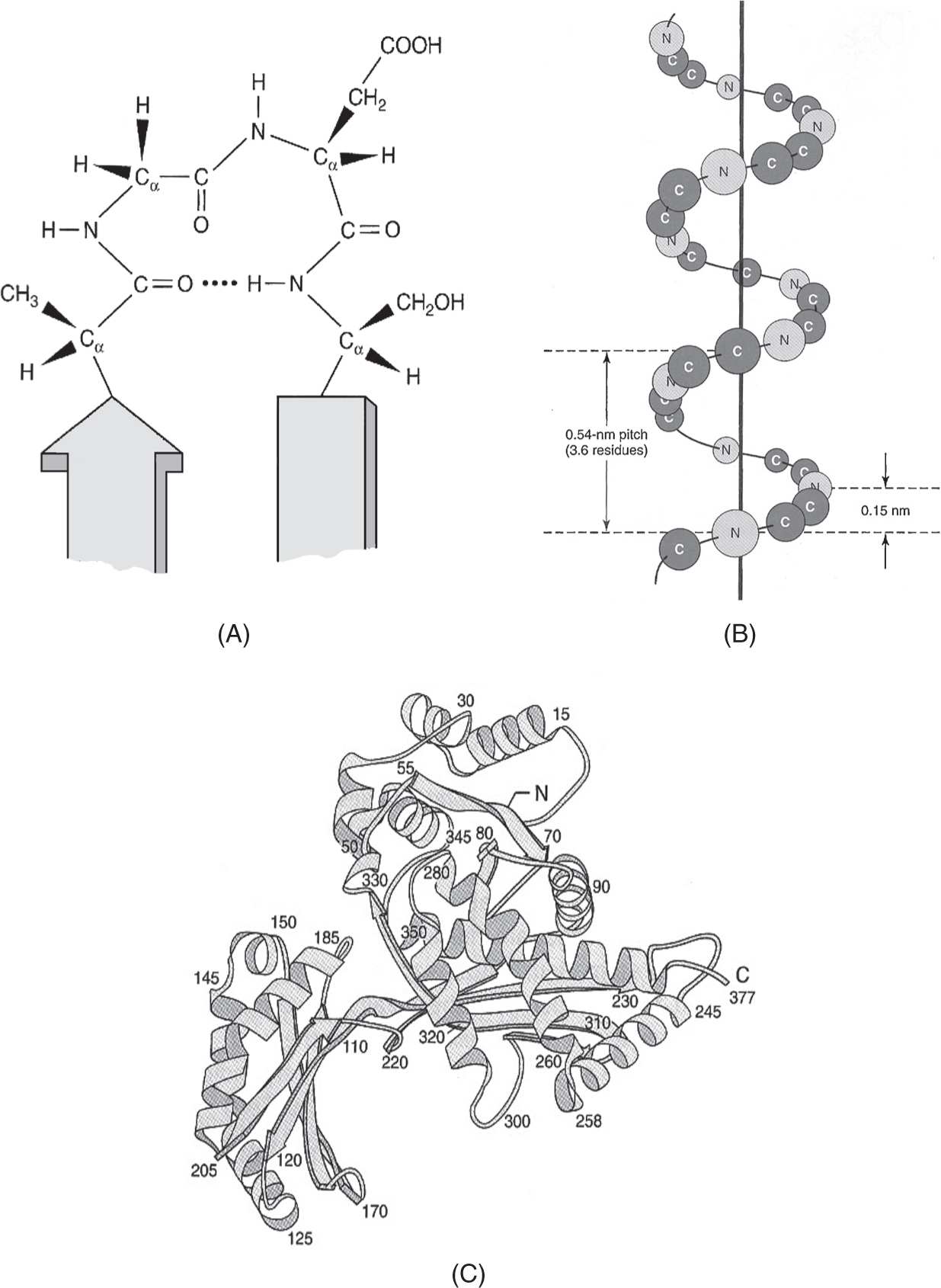
Figure 1
Levels of protein structure: (A) primary structure of O=C-NHC peptide bond (top center) shown as a turn within a secondary structure of antiparallel β sheets. The four-residue segment Ala-Gly-Asp-Ser is stabilized by a hydrogen bond (dotted line—center); (B) orientation of the core C and N atoms within a peptide segment with α-helical secondary structure; (C) tertiary structure of a bacterial enzyme illustrating the folding of sequential amino acid molecules (numbers) to form a three-dimensional protein scaffold with helical and β-sheet domains. (Reproduced, with permission, from Murray RK, Bender DA, Botham KM, et al. Harper’s Illustrated Biochemistry. 29th ed. New York, NY: McGraw-Hill; 2012:37-41.)
• Tertiary protein structures comprising one peptide chain can associate with other peptide chains (subunits) to form quaternary structures.
• Tertiary and quaternary structures are stabilized by hydrogen bonds (discussed earlier) and by covalent links such as disulfide (-S-S-) bonds.
PROTEIN STRUCTURE/FUNCTION
Key concepts: Protein structure/function and enzymes (Murray, pp 25-93. Scriver, pp 3-45. Lewis, pp 194-199.)
• The folding of proteins into tertiary and quaternary structures is step-wise, thermodynamically favored, and assisted by auxiliary proteins such as chaperones, disulfidases, and proline cis-trans isomerases.
• Protein conformation provides a diversity of protein functions illustrated by the fibrils of collagen or fibrillin, ligand binding like heme/bisphosphoglycerate with hemoglobin, and assembly of catalytic sites for enzymes.
• Influence of small molecules on protein conformation/function is exemplified by the Bohr effect on the hemoglobin saturation curve (Fig. 2) or initiation of signal transduction cascades by molecules such as cyclic adenosine monophosphate (cAMP).
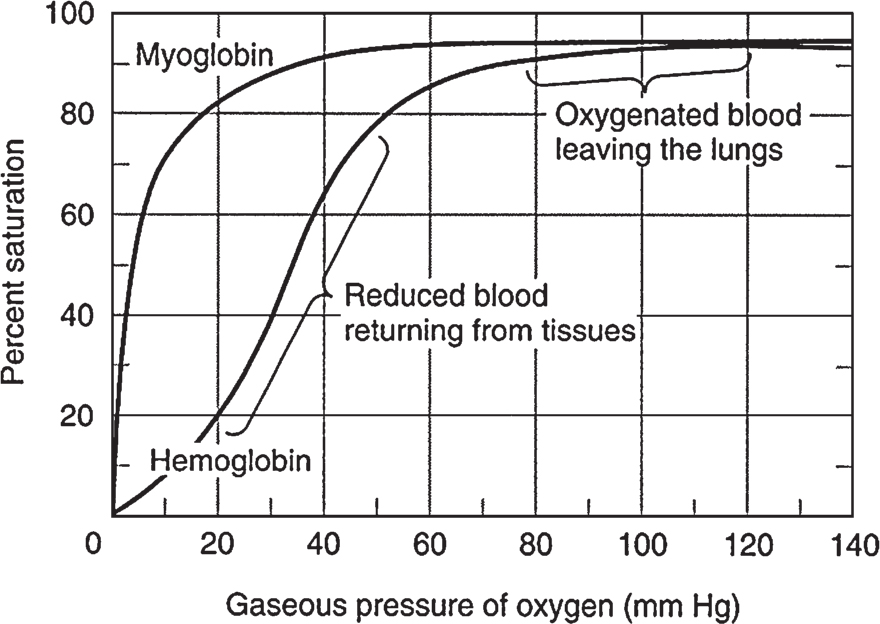
Figure 2
Oxygen binding curves of both hemoglobin and myoglobin. The arterial oxygen tension of blood leaving the lungs is about 100 mm Hg compared to 40 mm for veins returning blood from tissues or 20 mm within capillary beds of actively metabolizing tissues such as muscle. The contrast in percent oxygen saturation between hemoglobin (∼30%) and myoglobin (∼90%) in the 20 to 40 mm Hg oxygen tension region of the curve (the oxygen tension of peripheral tissues) illustrates that greater release of oxygen at low tensions made possible by the subunit structure of hemoglobin via cooperativity. (Reproduced, with permission, from Murray RK, Bender DA, Botham KM, et al. Harper’s Illustrated Biochemistry. 29th ed. New York, NY: McGraw-Hill; 2012:50.)
• Amounts of proteins may be measured by reaction with specific antibodies (eg, Western blotting or enzyme-linked immunosorbent assays [ELISA]) or, for enzyme proteins, by their rates of conversion of substrates to products (enzyme catalysis).
• Enzymes lower the activation energy for reactions of substrates (precursors) to products; the amino acid side groups within enzyme catalytic sites lower energy by forming substrate-enzyme intermediates (transition states).
• Enzyme assays use initial rate conditions with excess substrate such that the initial velocity (Vi) of substrate reaction is proportionate to enzyme concentration.
• For a given amount of enzyme, the relation between reaction velocity V and substrate concentration S is given by the Michaelis-Menton equation and its reciprocal (plotted in Fig. 3):
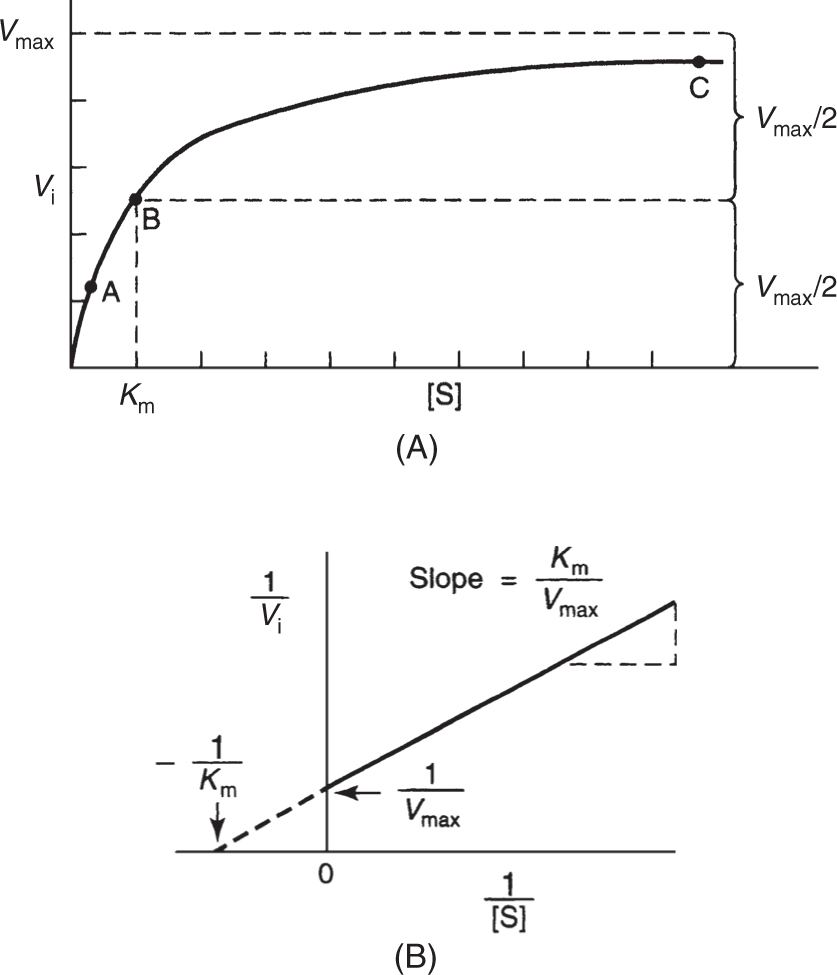
Figure 3
(A) Effect of substrate concentration on the initial velocity of an enzyme-catalyzed reaction. (B) Double reciprocal or Lineweaver-Burk plot of 1/Vi versus 1/[S] that can be used to evaluate Km and Vmax. (Reproduced, with permission, from Murray RK, Bender DA, Botham KM, et al. Harper’s Illustrated Biochemistry. 29th ed. New York, NY: McGraw-Hill; 2012:75-77.)

• The Michaelis constant Km is defined as the substrate concentration giving one-half the maximal reaction velocity, and can be viewed as a binding constant—substrates with high affinity for the enzyme catalytic site have low Km values and vice versa.
As S increases and becomes much greater than the Km, V approaches its maximal velocity Vmax:
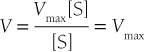
Substrates with higher affinity (lower Km) will thus saturate enzyme catalytic sites and achieve Vmax at lower concentrations.
• Because the Michaelis-Menton equation shows that attainment of Vmax is a hyperbolic curve (Fig. 3A), use of the reciprocal Lineweaver-Burk equation shown above provides an easy graphical determination of Vmax and Km as shown in Fig. 3B. The reciprocal of velocity 1/V is plotted on the y axis against the reciprocal of substrate concentration 1/S on the x axis. Direct graphic determination of Vmax is made by measuring the y intercept (= 1/Vmax when 1/S = 0). Direct graphic measurement of the K is made by measuring the x intercept (= ~1/K when 1/V = 0). The slope is Km/Vmax.
• The reciprocal Lineweaver-Burk plot constructed by measuring enzyme velocities with and without inhibitor distinguishes competitive inhibition from noncompetitive inhibition (Fig. 4). A competitive inhibitor will have similar structure to the substrate and compete for enzyme catalytic sites, changing the effective Km, but not the Vmax (Fig. 4A). A simple noncompetitive inhibitor does not resemble substrate in structure and will not compete for its binding at the enzyme catalytic site, binding instead to an allosteric site. Noncompetitive inhibitors thus change Vmax, but not Km (Fig. 4B).
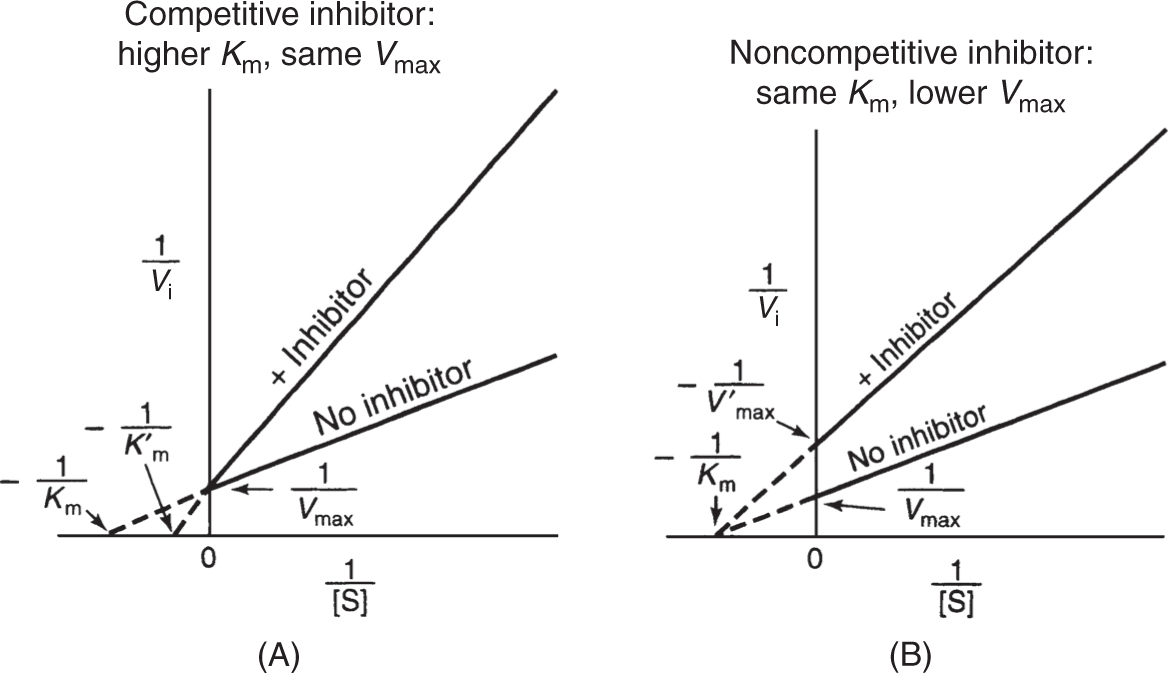
Figure 4
(A) Lineweaver-Burk plot of competitive inhibition. Note the complete relief of inhibition at high [S] (ie, low 1/[S] concentrations); (B) Lineweaver-Burk plot for simple noncompetitive inhibition. Note the change in Vmax with identical Km. (Reproduced, with permission, from Murray RK, Bender DA, Botham KM, et al. Harper’s Illustrated Biochemistry. 29th ed. New York, NY: McGraw-Hill; 2012:79.)
• Categories of enzymes include (See Table 2):
TABLE 2. CLASSES OF ENZYMES USED IN OXIDATION REACTIONS
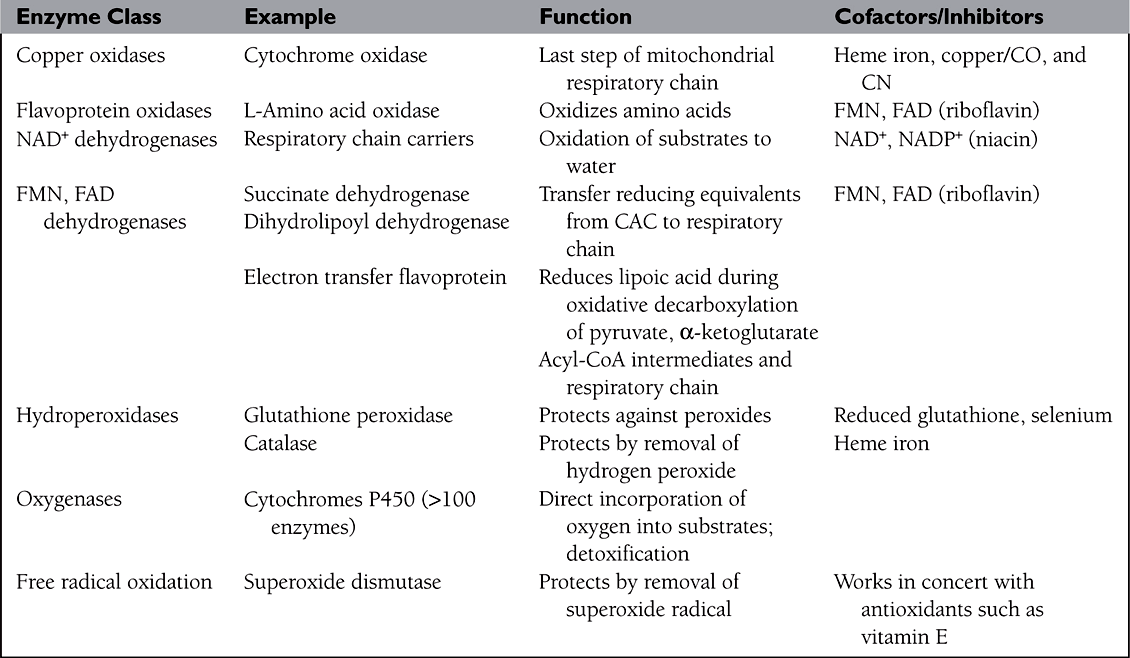
1. Oxidoreductases that add oxygen or remove hydrogens (oxidases, dehydrogenases) or add hydrogens (reductases) to substrates.
2. Transferases that move chemical groups (glycosyl, methyl, and phosphoryl) from one substrate to another.
3. Hydrolases that cleave substrate bonds using water (adding H or OH to the cleavage products); many subtypes including peptidases, lipases, etc.
4. Isomerases that rearrange substrate molecules to form a different isomer.
5. Synthases that join substrates by forming new bonds.
6. Phosphatases that remove phosphate groups and phosphorylases or kinases that add phosphate groups to substrates.
2. METABOLOME
INTERMEDIARY METABOLISM
Key concepts: Intermediary metabolism (Murray, pp 131-142. Scriver, pp 3-45.)
• Metabolism involves breakdown (catabolism) of dietary carbohydrates, fat, and protein to glucose, fatty acids/glycerol, and amino acids, respectively, providing energy for biosynthesis (anabolism) and organ function.
• Common metabolic diseases involve nutrient, vitamin, or hormone deficiencies that reflect interaction of genes and environment (multi-factorial determination), exemplified by marasmus, rickets, or diabetes mellitus.
• Rarer metabolic diseases (inborn errors of metabolism) result from specific enzyme deficiencies and exhibit autosomal or X-linked recessive inheritance; accumulation or deficiency of small molecules often produces acute catastrophic disease (acidosis, seizures, and coma), while accumulation of larger molecules often produces chronic disease (storage disease).
• The enzyme and cofactor deficiencies of inborn errors of metabolism can be defined in terms of substrate excess and product deficit, guiding diagnostic tests (blood amino acids, urine organic acids) and therapies (substrate avoidance and product or vitamin supplementation through the diet).
• Metabolic pathways are highly compartmentalized as enzyme complexes (eg, the pyruvate dehydrogenase with >30 peptides) or organelles (eg, citric acid cycle enzymes in the mitochondrial matrix); even the cytoplasm is a liquid crystal of heterogenous enzyme associations rather than a homogenous protein solution.
• The entry and flow (flux) of metabolites in pathways are controlled through compartmentalization, irreversibility of certain enzyme steps, binding of substrates by low Km, first-step pathway enzymes, and regulatory/rate-limiting enzymes that are activated or inhibited by small molecules (allosteric modifiers) or hormones.
CARBOHYDRATE AND GLYCOGEN METABOLISM
Key concepts: Carbohydrate structures and glycoproteins (Murray, pp 132-139. Scriver, pp 1407-1666.)
• Carbohydrates (sugars), and particularly glucose, are important metabolic fuels for mammals, particularly during fetal development.
• Glucose is the common currency of carbohydrate metabolism, synthesized in plant photosynthesis, ingested and absorbed into animal bloodstreams, and converted from other sugars in the liver (Fig. 5).
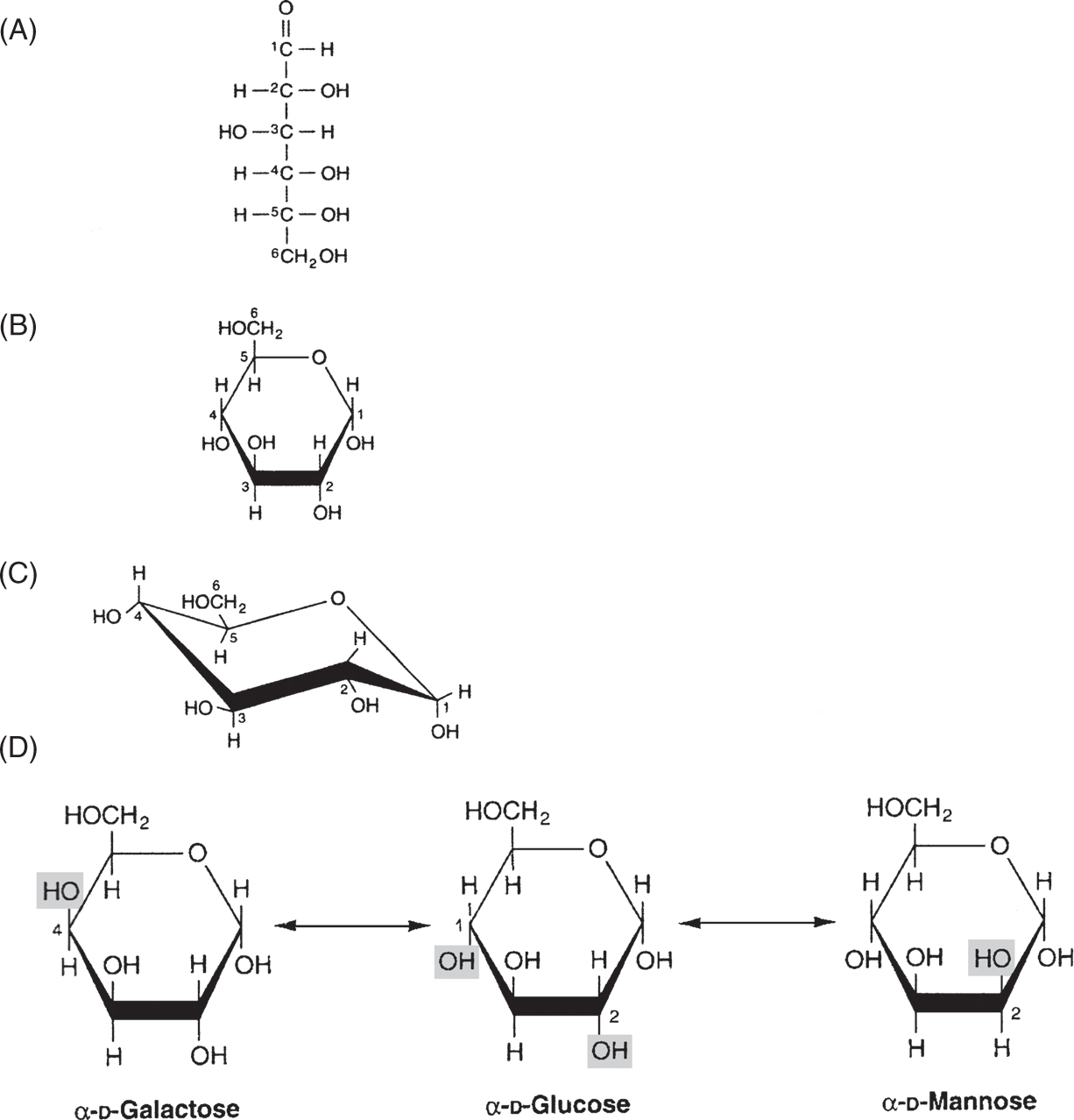
Figure 5
D-Glucose in (A) straight chain form; (B) with CI-O-C5 ring formation to yield CI hydroxyl orientation (arrow) as α-anomer; (C) actual structural conformation of α-D-glucose as Haworth projection showing -OH (and -CH2OH) groups in most stable position (in plane with ring) except for C1 α-hydroxyl (arrow—note that C1 β-hydroxyl would be in plane, accounting for 62% β, 38% α-anomers of D-glucose in water solution); (D) biologically important epimers of D-glucose shown as a-anomers (galactose and mannose) will include hydroxyls out of ring plane in Haworth projection, accounting for decreased stability and potential energy release when converted to glucose as “ground state” of animal metabolism. (Reproduced, with permission, from Murray RK, Bender DA, Botham KM, et al. Harper’s Illustrated Biochemistry. 29th ed. New York, NY: McGraw-Hill; 2012:133-134.)
• Monosaccharides include three-carbon trioses (glyceraldehyde), five-carbon pentoses (ribose, arabinose, and xylose), and six-carbon hexoses (glucose, galactose, and mannose); each group has many isomers (same structural formulas, but different arrangement of CHO groups).
• The four optically active carbons of hexoses with CH = O (aldehyde group) at carbon 1 (C1) produce 16 isomers including glucose; these include dextro (D) or levo (L) conformations at C4 (optical isomers), formation of four-carbon (furan) or five-carbon (pyran) rings through C1-O-C4 or C1-O-C5 bonds, and upward (β) or downward (α) conformations of the C1-hydroxyl group after ring formation (anomers).
• Important monosaccharides with a C = O group at C2 (ketoses) include fructose (six carbons), xylulose or ribulose (five carbons), and dihydroxy-acetone (three carbons).
• Disaccharides can be formed between various sugar isomers, including lactose (β–D-galactose-C1-O-C4-β–D-glucose = β–D-galactose-[1 → 4]-β-D-glucose), maltose (α–D-glucose-[1 → 4]-β–D-glucose), or sucrose (α-D-glucose-[1 → 2]-β-D-fructose).
• Longer sugar chains (polysaccharides) are composed of single sugars (eg, starch, amylopectin, and glycogen with α–D-glucose 1 → 4 links and 1 → 6 branches) or multiple sugars (eg, mucopolysaccharides or heparin with glucuronic acid, acetylglucosamine, iduronic acid groups, etc).
• Sugars may be added to amino acid side chains on proteins to form glycoproteins, illustrated by influenza virus hemagglutinins, coagulation proteins (such as thrombin), surface antigens (such as the ABO blood groups), or glycosylated hemoglobin A1c formed during hyperglycemia from uncontrolled diabetes mellitus. The latter modification is extremely important in monitoring diabetic control and outcomes, since the 120-day life span of red blood cells and their component hemoglobin reflects average glucose levels over that time period.
Key concepts: Carbohydrate metabolism (Murray, pp 131-139, 170-206. Scriver, pp 1407-1666.)
• Major themes of human carbohydrate metabolism include the distribution of glucose to tissues, metabolism to lactate in anaerobic tissues, metabolism to pyruvate and acetyl coenzyme A (acetyl-CoA) in aerobic tissues, and generation of energy (ATP) through the citric acid cycle and oxidative phosphorylation (ox-phos).
• Glucose, fructose, and galactose are the main carbohydrates absorbed from dietary starch, sucrose, and lactose, respectively; fructose and galactose are converted to glucose in liver (mainly) and deficiencies in their conversion cause essential fructosemia and galactosemia.
• Glycolysis (Fig. 6) is the main path of glucose metabolism (and of dietary fructose or galactose), producing lactate in anaerobic tissues (brain, muscle, gastrointestinal tract, retina, skin, and erythrocytes with no mitochondria).
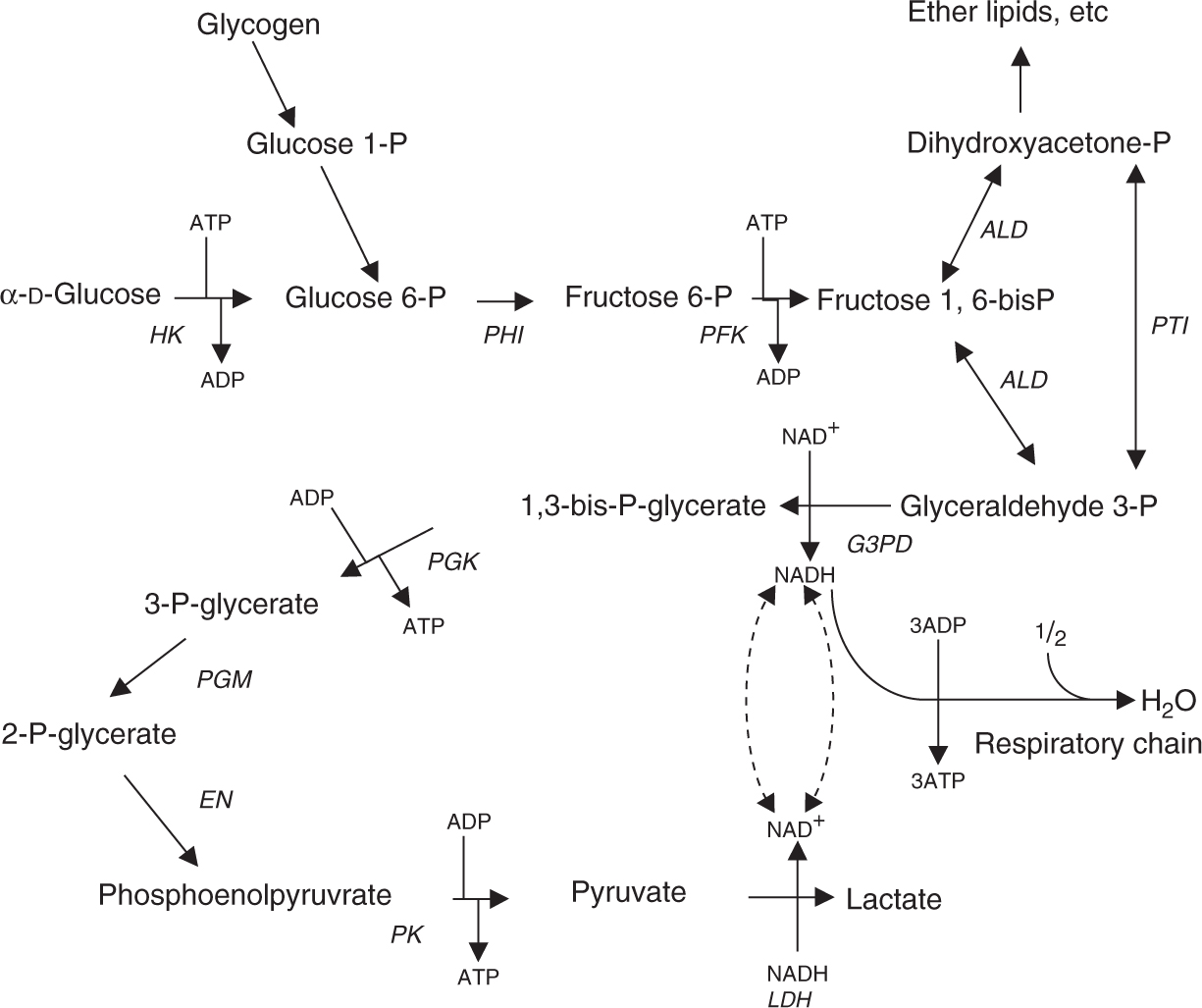
Figure 6
Pathway of glycolysis showing steps yielding ATP (adenosine triphosphate) and NADH (nicotinamide dinucleotide), which is subsequently oxidized in the mitochondrial respiratory chain. P, phosphoro- or phosphate; enzymes in italics: HK, hexokinase; PHI, phosphohexose isomerase; PFK, phosphofructokinase; ALD, aldolase; PTI, phosphotriose isomerase; G3PD, glyceraldehyde-3-phosphate dehydrogenase; PGK, phosphoglycerate kinase; PGM, phosphoglycerate mutase; EN, enolase; PK, pyruvate kinase; LDH, lactate dehydrogenase. (Modified, with permission, from Murray RK, Bender DA, Botham KM, et al. Harper’s Illustrated Biochemistry. 29th ed. New York, NY: McGraw-Hill; 2012:172.)
• Aerobic tissues such as heart, liver, or kidney glycolyze glucose to pyruvate, convert pyruvate to acetyl-CoA via pyruvate dehydrogenase (PDH), and effect complete oxidation to carbon dioxide and water using the citric acid cycle (CAC) and ox-phos.
• Aerobic tissues oxidize lactate when oxygen is plentiful and ox-phos is effective, but produce lactic acidosis under circumstances of hypoxia, cardiopulmonary failure, exercise fatigue, or ox-phos interruption (PDH or thiamine cofactor deficiencies), PDH inhibitors (arsenate, mercurate ions), mitochondrial diseases, and alcoholism with thiamine deficiency.
• Deficiencies of glycolytic enzymes have predominant effects in anaerobic tissues including hemolytic anemias or myopathies.
• The pentose phosphate pathway is an alternative route for glucose metabolism that generates reducing equivalents of NADPH for steroid/fatty acid synthesis and ribose for nucleotide formation.
• The citric acid cycle (CAC) is a final common pathway for carbohydrate, lipid, and amino acid metabolism, combining acetyl-CoA with oxaloacetate and cycling through seven intermediates to generate oxaloacetate again (Fig. 7).
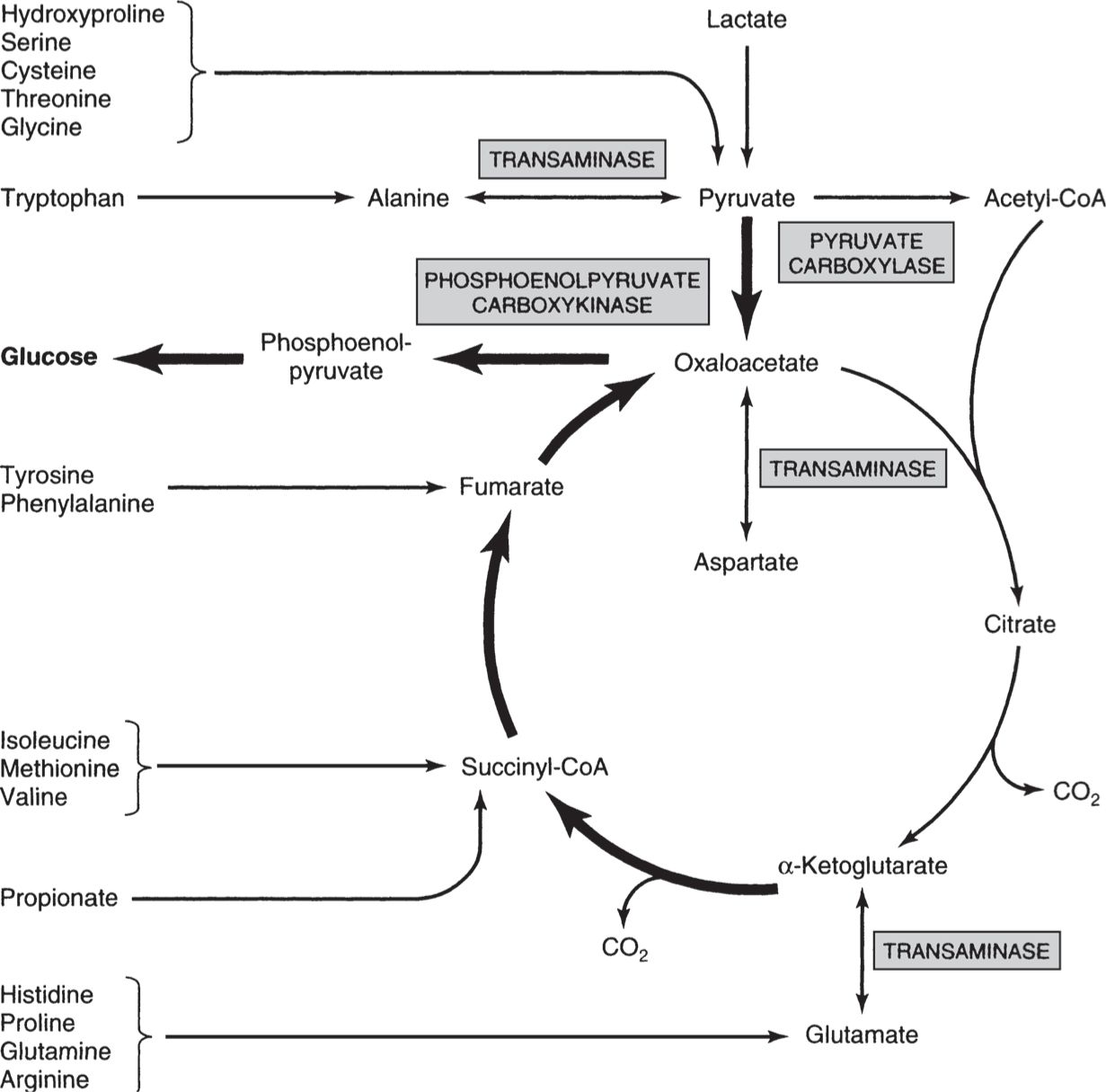
Figure 7
Involvement of the citric acid cycle in transamination and gluconeogenesis. Bold arrows indicate the main pathway of gluconeogenesis. (Reproduced, with permission, from Murray RK, Bender DA, Botham KM, et al. Harper’s Illustrated Biochemistry. 29th ed. New York, NY: McGraw-Hill; 2012:167.)
• The CAC is channeled within mitochondria, directly generating ATP or substrates such as reduced nicotinamide-NADH or flavin adenine (FADH2) dinucleotides that produce ATP through ox-phos.
• The CAC is amphibolic, involved in catabolism to yield ATP energy and anabolism through gluconeogenesis and fatty acid synthesis.
• The CAC is under respiratory control, being tightly coupled to ox-phos by levels of oxidized cofactors (such as NAD+) and by end-product inhibition of PDH (acetyl-CoA, NADH).
Key concepts: Glycogen metabolism and gluconeogenesis (Murray, pp 178-190. Scriver, pp 1521-1552.)
• Glycogen is stored glucose in the form of a branched polysaccharide analogous to starch in plants; it accounts for 6% of liver mass and 1% of muscle mass in fed states.
• Glycogenesis utilizes high-energy uridine diphosphate glucose (UDPGlc) formed from glucose 1-phosphate and UTP, forming 1 → 4 links with a synthase and 1 → 6 links with branching enzymes.
• Glycogen synthase adds glucose units to preexisting glucose chains on the primer protein glycogenin; the primer glucose chain is attached to the hydroxyl of a tyrosine residue.
• Glycogenolysis is very different from glycogen synthesis, requiring multiple enzymes such as glycogen phosphorylase that employs phosphate to hydrolyze 1 → 4 links and debranching enzymes that hydrolyze 1 → 6 links. Liberated glucose 1-phosphate is converted to glucose 6-phosphate and (in liver) to glucose by glucose-6-phosphatase (thus increasing blood glucose—Fig. 8).
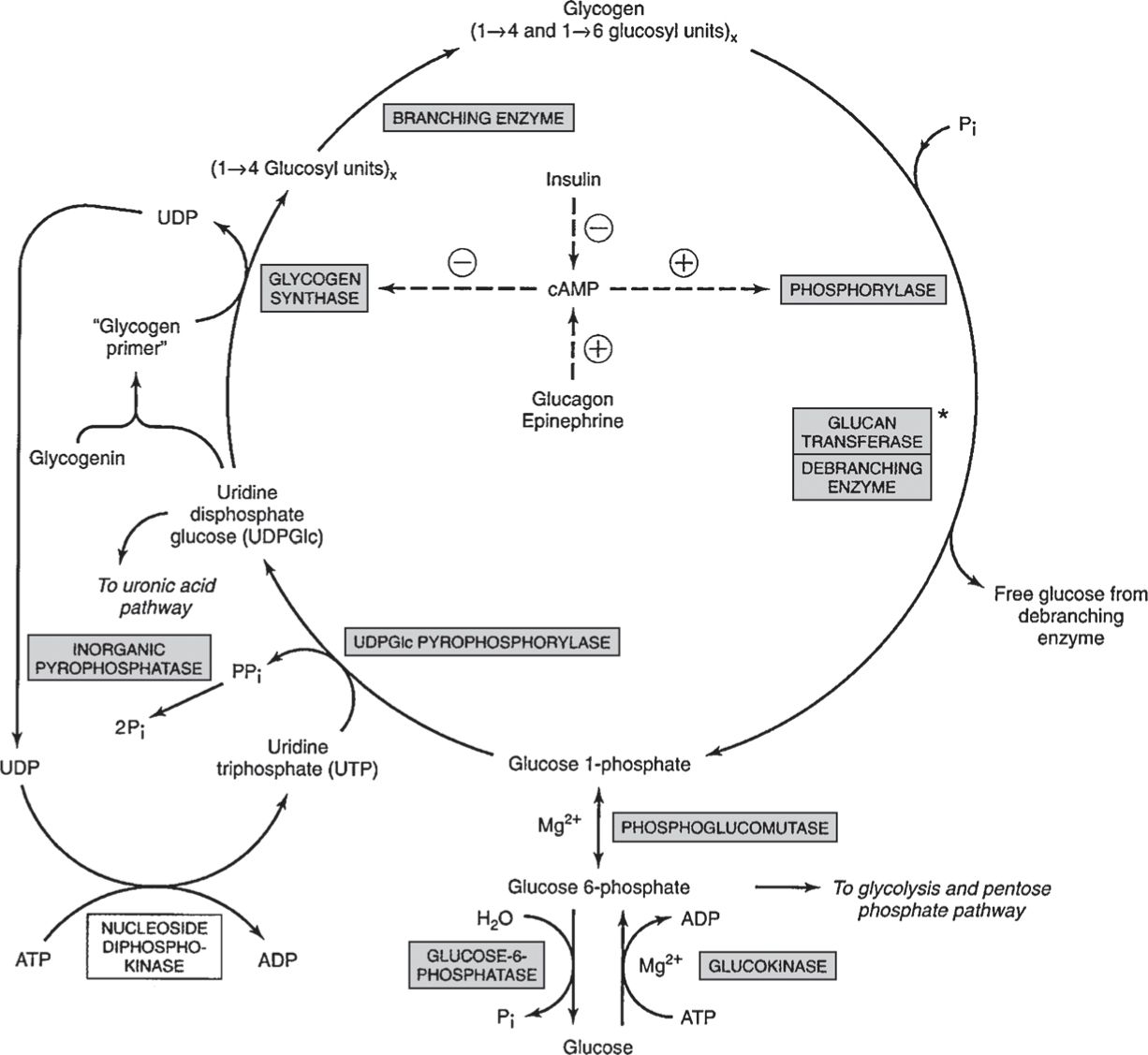
Figure 8
Pathway of glycogenesis and glycogenolysis in the liver. Two mol of high-energy phosphates are used in the incorporation of 1 mol of glucose into glycogen. + refers to simulation, –to inhibition. Insulin decreases the level of cAMP only after it has been raised by glucagons or epinephrine—that is, it antagonizes their action. Glucagon is active in heart muscle but not in skeletal muscle. At asterisk; Glucan transferase and debranching enzyme appear to be two separate activities of the same enzyme. (Reproduced, with permission, from Murray RK, Bender DA, Botham KM, et al. Harper’s Illustrated Biochemistry. 29th ed. New York, NY: McGraw-Hill; 2012:179.)
• Phosphorylases differ between liver and muscle, activated by phosphorylation of their serine hydroxyl groups (via phosphorylase kinase and protein phosphatase) and by allosteric interactions with cyclic AMP or AMP.
• Cyclic AMP is formed from ATP by adenyl cyclase in response to hormones such as epinephrine, norepinephrine, and glucagon; it is hydrolyzed by phosphodiesterase to produce 5′ AMP, an activator of muscle phosphorylase during fatigue (Fig. 8).
• Insulin slows glycogenolysis by direct stimulation of liver phosphodiesterase (to decrease cAMP) and inhibition of liver glycogen phosphorylase through glucose uptake, increased formation of glucose 6-phosphate, and reduced activity of phosphorylase kinase.
• Inherited enzyme deficiencies produce glycogen storage in liver or muscle according to enzyme location (Table 3); failure of liver glycogenolysis and blood glucose supply cause recurrent hypoglycemia, lactic acidemia, and shift to fatty acid metabolism with hypercholesterolemia and hyperuricemia.
TABLE 3. GLYCOGEN STORAGE DISEASES
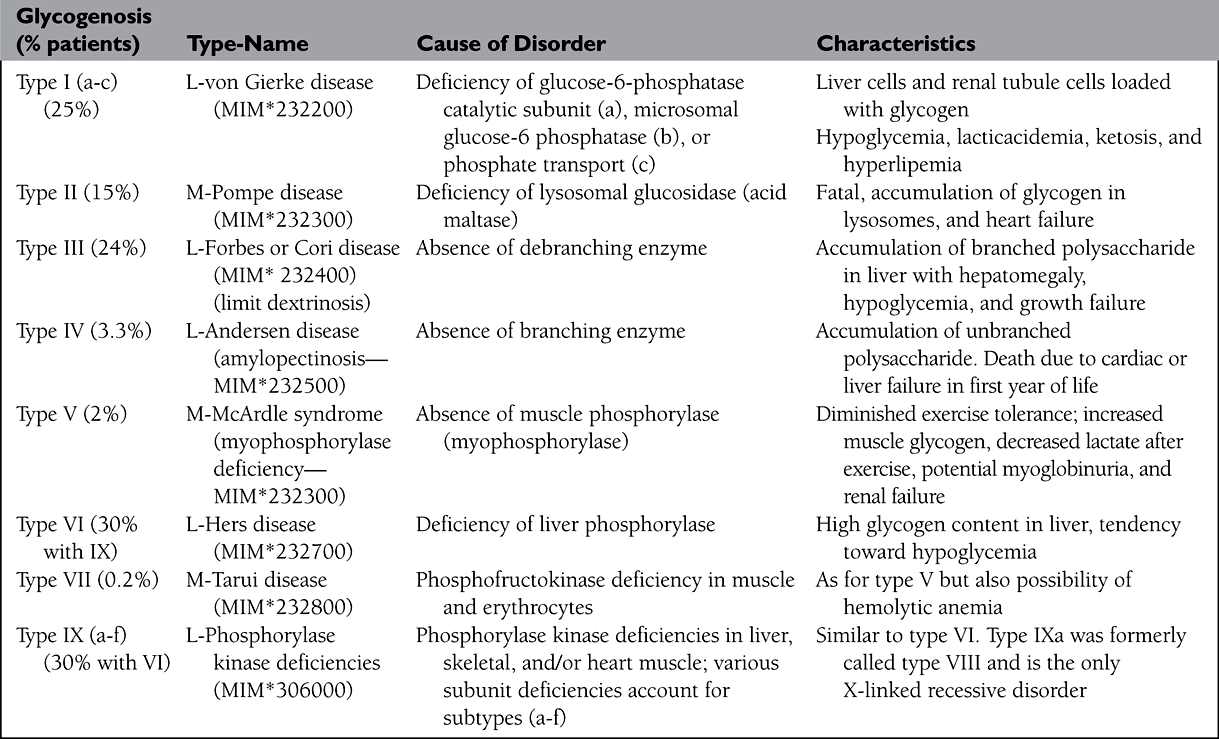
BIOENERGETICS, ENERGY METABOLISM, AND BIOLOGICAL OXIDATION
Key concepts: Bioenergetics and energy metabolism (Murray, pp 109-114. Scriver, pp 2261-2296.)
• Bioenergetics is the study of energy changes that accompany metabolism; biological systems use chemical energy, taking fuel from food.
• Food and micronutrient (vitamins, minerals) availability determine the rate of energy release, modulated by thyroid hormones.
• States of energy depletion (starvation, marasmus) or excess (obesity) are powerful influences in medicine and disease.
• Biochemical reactions are of two types—exergonic (catabolic) that yield energy (eg, conversion of phosphoenolpyruvate to pyruvate) and endergonic (anabolic) that consume energy (eg, conversion of glucose to glucose 6-phosphate and synthesis of biopolymers). Exergonic reaction products (eg, NADH, ATP) are often coupled to endergonic (synthetic) reactions (eg, production of ATP, GTP during fat or glucose oxidation, and GTP hydrolysis during aminoacyl translocation of protein synthesis).
• High-energy phosphates are the currency of cellular energy exchange, including ATP and GTP; glycolytic compounds 1,3-bisphosphoglycerate and phosphoenolpyruvate; and creatine phosphate in muscle.
• Other high-energy compounds include coenzyme A derivatives, S-adenosylmethionine, and UDP-glucose.
• The ratio of ATP to ADP reflects cellular energy potential and coordinates various processes such as oxidative-phosphorylation, glycolysis, and the citric acid cycle.
• Enzymes such as myokinase or adenyl kinase catalyze ATP-ADP-AMP interconversions in response to cellular energy supplies.
Key concepts: Biological oxidation (Murray, pp 115-120. Scriver, pp 2261-2296.)
• Chemical oxidation of a substrate involves removal of electrons, while chemical reduction involves a gain in electrons.
• Oxygen provided by animal respiration provides direct oxidation (addition of oxygen to substrates by oxidases, cytochromes P450, etc) or indirect oxidation (removal of hydrogens from substrates to form water by dehydrogenases, etc).
• The generation of energy by oxidation-reduction reactions is proportionate to their redox potential (analogous to battery voltage); the conversion of oxygen to water (last step of the respiratory chain) has the greatest (most positive) redox potential.
• The high redox potential of oxygen to water conversion drives the intermediate reactions of food oxidation/metabolism, generating reducing equivalents that are converted to fuel (ATP, high-energy phosphates) by ox-phos.
• Several classes of enzymes catalyze oxidative reactions, using the redox potential to generate high-energy compounds or to detoxify drugs or environmental agents (see Table 2).
LIPID, AMINO ACID, AND NUCLEOTIDE METABOLISM
Key concepts: Significant lipids and lipid synthesis (Murray, pp 140-150, 216-228. Scriver, pp 2705-2716.)
• Lipids, including fats, oils, steroids, and waxes, are related by their insolubility in water and function in energy storage, membrane barriers, and neural insulation.
• Simple lipids are fatty acids linked with alcohols to form esters, including fats (solid glycerol esters), oils (liquid glycerol esters), and waxes (fatty acids linked to long-chain alcohols).
• Complex lipids include links to phosphoric acid (phospholipids), the 18-carbon amino alcohol sphingosine (sphingophospholipids), carbohydrate (glycosphingolipids), and proteins (including lipoproteins).
• Derived lipids include saturated (no double bonds) or polyunsaturated fatty acids (multiple double bonds), polyunsaturated fatty acids with rings (eicosanoids including prostaglandins and leukotrienes, vitamins including E and K), steroids derived from cholesterol including hormones (aldosterone, estrogen, and testosterone) or vitamins (vitamin D), and polymers containing ceramide (a sphingosine derivative), carbohydrates (generating cerebrosides), or carbohydrates with sialic acid (gangliosides).
• Amphipathic lipids (eg, glycerophospholipids) contain hydrophobic (water-repelling) and hydrophilic (water-soluble) regions; they form water-lipid interfaces such as bilayer membranes or multilamellar sheaths, micelles (droplets with oil inside), and liposomes (water inside).
• Fatty acid synthesis begins in the cytoplasm with biotin-mediated carboxylation of acetyl-CoA to form malonyl-CoA by acetyl-CoA carboxylase (Fig. 9). Initial fatty acyl synthesis is sequestered within a dimeric, 14-enzyme fatty acid synthase complex that transfers the CoA molecules onto pantothenic acid sulfhydryl groups before condensing them to form enzyme-bound acetoacetyl groups.
Figure 9
Synthesis and elongation of fatty acids. Initial synthesis of a fatty acid occurs within a fatty acid synthase complex composed of two symmetrical dimmers, each with seven enzymes plus pantothenic acid. Acetyl-CoA is carboxylated using biotin to yield malonyl-CoA, and each molecule is transferred to apposing pantothenic acid sulfhydryl groups within the synthase followed by condensation to make acetoacetyl-CoA (R = H indiagram). Elongation of fatty acids above 10 carbons is performed by a microsomal fatty acid elongase that condenses malonyl and acetyl-CoA directly, followed by similar steps of reduction to hydroxyacyl-CoA, enoyl-CoA, and acyl-CoA. Each cycle of this reaction sequence adds two carbons to the fatty acyl chain, occurring within the synthase complex for C4-C16 and/or within microsomes for C10 and above. (Modified, with permission, from Murray RK, Bender DA, Botham KM, et al. Harper’s Illustrated Biochemistry. 29th ed. New York, NY: McGraw-Hill; 2012:218.)
• A microsomal fatty acid elongase extends C10 and longer fatty acids through direct condensation of fatty acyl- and acetyl-CoA. Fatty acids are thus made using acetyl-CoA building blocks, with similar steps of two-carbon addition for the synthase or elongation pathways (Fig. 9).
• Linoleic (C18 with two double bonds), α-linolenic (C18 with three double bonds), and arachidonic acids (C20 with four double bonds and precursor to prostaglandins) cannot be synthesized in humans and must be supplied in the diet (essential fatty acids); essential fatty acid deficiencies can occur with intestinal malabsorption (cystic fibrosis, Crohn disease) or liver disease (cirrhosis, alcoholism, and immature neonatal liver), while excess trans-unsaturated fatty acids are associated with atherosclerosis.
• Glycerophospholipids (ie, lung surfactant, cholines, and inositol second messengers) are synthesized from acyl-CoAs (activated by acyl-CoA synthetase) and glycerol-3-phosphate, while glycerol-ether lipids (platelet activating factor) are analogously synthesized from dihydroxyacetone phosphates.
• Fatty acid synthesis (lipogenesis) is promoted in fed states through supplies of NADPH from the pentose phosphate pathway, allosteric activation of acetyl-CoA carboxylase by citrate (high concentrations when acetyl-CoA is abundant), and inhibition of acetyl-CoA carboxylase and pyruvate dehydrogenase by the products of lipogenesis, long-chain fatty acyl-CoAs.
• Insulin increases lipogenesis by inhibiting phosphorylation and inactivation of acetyl-CoA carboxylase, while epinephrine and glucagon inhibit lipogenesis and promote lipolysis; insulin also decreases cellular cAMP, reducing lipolysis in adipose tissue and decreasing plasma concentrations of free fatty acids.
Key concepts: Lipid catabolism and transport (Murray, pp 207-215. Scriver, pp 2705-2716.)
• Glycosphingolipid polymers such as ceramides or gangliosides are degraded by lysosomal enzymes that, when deficient, cause lipid storage diseases affecting brain, bones, and the reticuloendothelial system (Tay-Sachs, Gaucher, and Niemann-Pick diseases—see table in Appendix for disease descriptions).
• Glycerophospholipids are degraded by phospholipases, some of which occur in snake venom; the combined action of phospholipases with fatty acyl activation and transfer allows remodeling of glycerol lipids through exchange of fatty acid groups.
• Fatty acid oxidation occurs in mitochondria, utilizing NAD+, FAD, and oxygen to remove two-carbon blocks and generate ATP (Fig. 10).
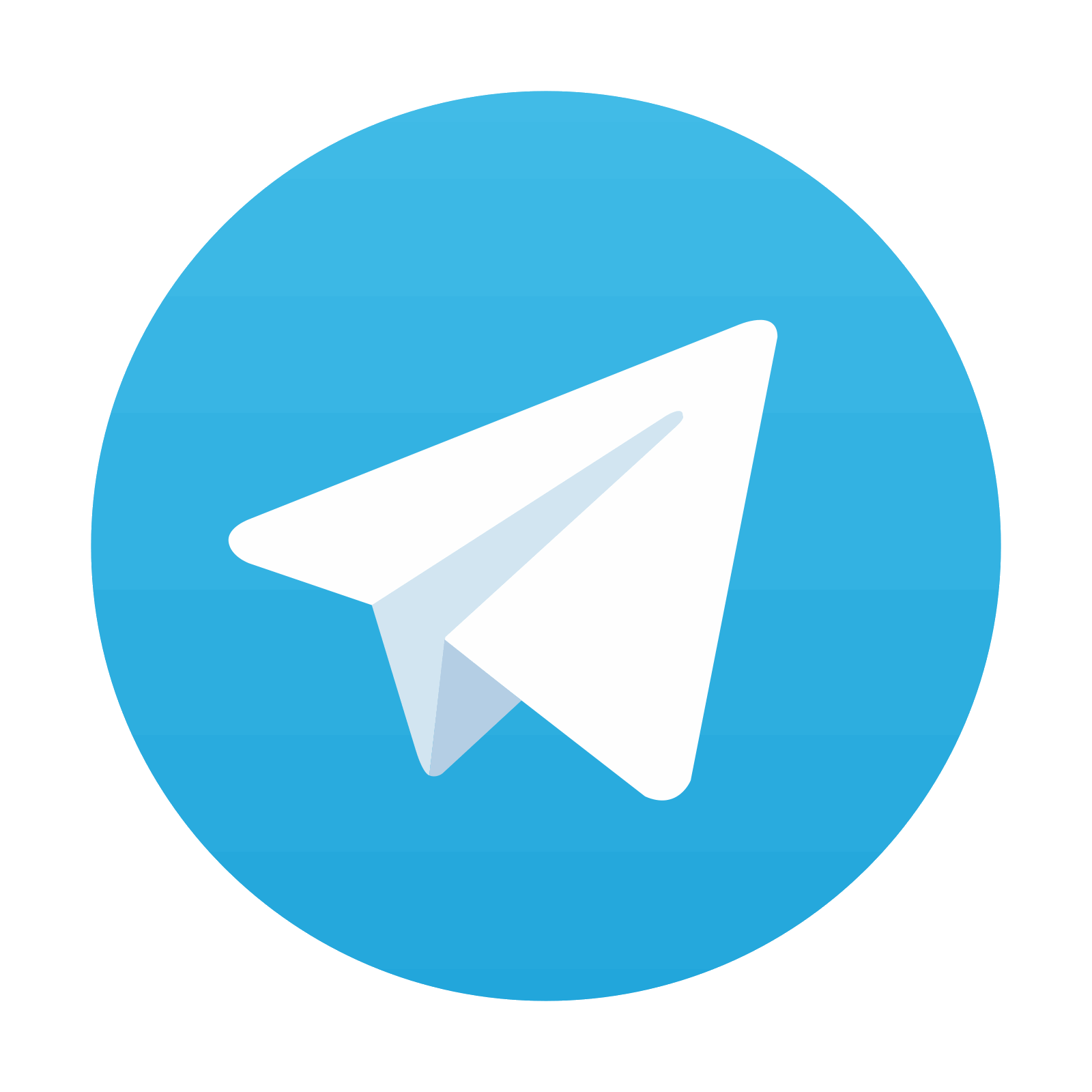
Stay updated, free articles. Join our Telegram channel
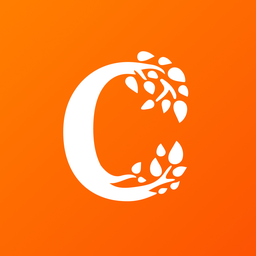
Full access? Get Clinical Tree
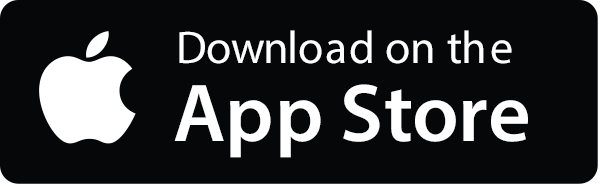
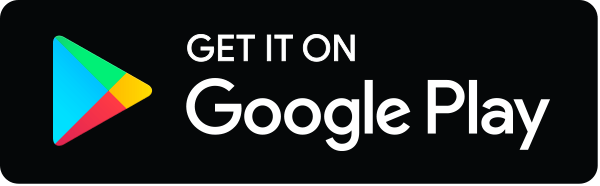