Fig. 15.1
Representative DNA nanostructures. (a) Representative DNA double crossover, known as DAE (double crossover in antiparallel with even number of helical half turns between crossovers) (Lin et al. 2009; Seeman 1982) (Adapted with permission from (Lin et al. 2009), copyright (2009) American Chemical Society). (b) Four-way DNA tile built of Holliday junction-like DNA junctions, which may assemble into two-dimensional DNA arrays as shown in (c) (Adapted from (Yan et al. 2003). Reprinted with permission from AAAS). (d) DNA origami assembled from about 7 kilobase circular M13 genomic DNA that is folded by about 200 short DNA “staple” strands (From (Rothemund 2006). Reprinted with permission from AAAS). (e) Tetrahedral-shaped DNA (Reproduced with permission from (Bath and Turberfield 2007)). (f) Curved DNA bundle with non-bent teeth, four of which assembles into a twelve-tooth gear (From (Dietz et al. 2009). Reprinted with permission from AAAS). (g) Vase-shaped DNA origami with curvature (From (Han et al. 2011). Reprinted with permission from AAAS)
Aside from regular double-strand DNA in plasmid or other transfectant vectors, DNA nanostructures are more compact, which may facilitate their cellular uptake and may help enhance nuclease resistance due to steric hindrance (Keum and Bermudez 2009; Mei et al. 2011). There are many reports demonstrating the feasibility of DNA nanostructures as nano-vehicles for therapeutic cargo transportation ranging from imaging agents and chemical compounds (Jiang et al. 2012; Kim et al. 2013; Li et al. 2013a; Zhang et al. 2014; Yan et al. 2015) to functional nucleic acid motifs (e.g., aptamer, antisense oligonucleotides, siRNA, miRNA, and CpG ODN) (Keum et al. 2011; Li et al. 2011; Schüller et al. 2011; Mohri et al. 2012; Lee et al. 2012). Furthermore, changes in pH or cation concentration within the cellular environment may trigger conformational changes of DNA motifs, forming such structures as i-motifs and G-quadruplexes, which, when used in combination with various DNA nanostructures, has proven to be effective for controlled release of drugs in anticancer therapy (Yang et al. 2012; Dong et al. 2014; Li and Famulok 2013).
Composed of mostly natural biological components, DNA nanostructures are considered to be biocompatible. Despite the enhanced nuclease resistance of DNA nanostructures compared to conventional double-stranded DNA (Keum and Bermudez 2009; Mei et al. 2011), they are still susceptible to degradation in biological systems, due both to nuclease digestion and component strand dissociation caused by changes in cation concentrations (Hahn et al. 2014). While such biodegradable features reduce the risk of DNA-associated toxicity during prolonged circulation, sufficient stability within the nanostructure is required to carry out the designed biological function. Thus, new efforts are needed to design and program DNA nanostructures with the expressed purpose of exhibiting optimal stability, a feature critical to the proper function of DNA-based nanomaterials in vivo.
15.3 Immune Stimulatory Activity of DNA Molecules and Their Feedback Regulation
In a live eukaryotic cell, DNA molecules outside their natural nuclear or mitochondrial compartments are considered PAMPs, which alert the host cell to an invasion by microbial pathogens or the presence of damaged cells (Barber 2011; Desmet and Ishii 2012). PAMPs function as potent activators of the innate immune system, resulting in production of a battery of proinflammatory cytokines, leading to the removal of infectious microbes or the clearance of damaged cells (Kumar et al. 2011). Two major signaling pathways have been identified to sense DNA “danger” signals and transduce them into sterile inflammation (Wu and Chen 2014). Unmethylated CpG motifs, which are common to bacterial and viral DNA but rarely found in the mammalian genome, are major ligands of TLR9 localized in the endoplasmic reticulum (Vollmer and Krieg 2009). Upon interaction with the internalized CpG DNA, TLR9 is translocated to the endolysosomal compartment, activating the myeloid differentiation primary response 88 (MYD88)-mediated signaling pathway and resulting production of type I interferon (IFN) and other proinflammatory cytokines (Latz et al. 2004). This pathway operates primarily in phagocytic and antigen-presenting cells (APCs) and serves as the critical host defense system against virus and bacteria. However, the DNA-mediated signaling pathway that controls IFN production was also found in cells lacking TLR9, such as many non-immune cell types, in which intracellular DNA devoid of the CpG motif still bears immune stimulatory activity (Ishii and Akira 2006; Vilaysane and Muruve 2009). Several DNA receptors have been identified in various cell types. Recently, it has been discovered that the signaling cascade of these DNA sensors is mediated by an adaptor protein, known as stimulator of interferon genes (STINGs), which leads to the production of type I IFN (IFN-alpha) (Abe et al. 2013; Konno and Barber 2014). Furthermore, cyclic GMP-AMP synthase (cGAS) was identified as a non-redundant and universal cytosolic DNA sensor (Wu et al. 2013; Li et al. 2013b). Upon binding to DNA, cGAS catalyzes the conversion of ATP and GTP into cyclic GMP-AMPs (cGAMP), which function as a second message to activate STING (Wu et al. 2013). The cGAS-cGAMP-STING signaling pathway is functional in many different cell types.
In addition to rendering immediate innate immunity against microbial DNA and damaged endogenous genome, the inflammation induced by both TLR9 and STING signaling pathways helps activate the body’s adaptive immunity against those targets. For the past two decades, CpG DNA has been extensively developed as a vaccine adjuvant to increase immunity against cancer and infectious agents (Vollmer and Krieg 2009; Murad and Clay 2009). Recently, it has been demonstrated that dendritic cells activate the STING signaling pathway to induce antitumor immunity by sensing DNA derived from apoptotic tumor cells upon irradiation or chemical therapy (Deng et al. 2012; Woo et al. 2014). Thus, similar to TLR9 ligands, extracellular DNA molecules, possibly including synthetic DNA nanostructures, could also be explored as adjuvants to induce both innate and adaptive immunities.
Although type I IFN is critical to host defense against invading pathogens, its excessive production can cause inflammation and provoke autoimmune diseases (Choubey 2012), such as systemic lupus erythematosus (SLE). To avoid this dire consequence, mammalian cells are equipped with several regulatory mechanisms to ensure that the inflammatory pathway is activated only transiently. First, both foreign DNA and self-DNA released from nuclear or mitochondrial compartments are usually degraded rapidly by several DNA-degrading nucleases, including DNAse I, DNAse II (lysosomal endonuclease), and DNAse III (3’ repair exonuclease, also known as Trex1). Defects in DNAse I and III have been associated with autoimmune diseases (Ablasser et al. 2014; Tsukumo and Yasutomo 2004), while DNAse II-deficient mice die late in embryogenesis, due to overproduction of type I IFN (Ahn et al. 2012; Yoshida et al. 2005). Second, both TLR9-MYD88 and cGAS-cGAMP-STING signaling pathways are stringently regulated to prevent overt activation. For example, type I/II IFN has been shown to induce indoleamine-2, 3-dioxygenase (IDO), an enzyme that catalyzes oxidative catabolism of tryptophan into kynurenine (Puccetti 2007). By depleting tryptophan, an essential amino acid, and producing kynurenine, IDO exerts metabolic immune regulation, promoting tolerance and immunosuppression (Grohmann et al. 2003). Thus, the IDO-mediated negative regulation may be intrinsically coupled to the DNA-sensing pathways to enforce homeostasis of immune stimulation and tolerance (Munn and Mellor 2013; Lemos et al. 2014). This regulation ensures a transient activation of innate immunity in response to extracellular DNA. Therefore, although multiple DNA-sensing pathways promote an immediate containment of invasion as well as long-term protection against pathogens, they are tightly regulated from overreaction, therefore minimizing the risk of DNA-mediated autoimmunity. Conceivably, exogenous DNA, such as those widely tested in gene therapy and vaccination, may bind to various DNA sensors for TLR9-dependent and cGAS-cGAMP-STING-mediated signaling pathways and trigger a transient activation of the innate system, imposing minimal toxicity in a host with functional nucleases and a DNA regulatory mechanism. This argument is in line with the general safety record of DNA vaccines tested in vivo, including both animal and clinical studies (Stenler et al. 2014; Hayton et al. 2014).
DNA nanostructures are readily taken up by macrophages and dendritic cells (Li et al. 2013a; Liu et al. 2012). The internalized DNA molecules were often found within endolysomal compartments (Mohri et al. 2012; Liu et al. 2012), which are likely sensed by the TLR9 signaling pathway for the activation of APCs. We and many other groups have shown that DNA nanostructures assembled with multiple copies of CpG demonstrated potent stimulation of macrophages and dendritic cells (Liang et al. 2014; Li et al. 2011; Mohri et al. 2012). Thus, DNA nanostructures can be customized by incorporation of CpG for enhanced adjuvant activity, which has been harnessed for anticancer therapy (Nishikawa et al. 2010, 2011; Kim et al. 2013; Zhang et al. 2014; Lee et al. 2012) and vaccine development (Liu et al. 2012; Rattanakiat et al. 2012; Nishikawa et al. 2014). Meanwhile, DNA-mediated activation should be controlled at an appropriate level and duration to avoid persistent inflammation and autoimmune reaction.
15.4 DNA-Nanoscaffolded Vaccines
Owing to robust engineering capability and intrinsic adjuvant activity, DNA represents an ideal platform for assembling both antigen and adjuvants on the same complex. With their particulate structures, the assembled DNA vaccine particles, like many nanomaterial-based vaccines, have the potential to facilitate the antigen delivery and antigen retention in the secondary lymphoid tissues and mediate co-delivery of both antigen and adjuvants to antigen-presenting cells. This functionality is critical to the initiation of adaptive immunity. For example, as reported recently by Nishikawa et al., a model antigen, ovalbumin (OVA), upon incorporation via physical trapping into self-assembled CpG nanoparticles or DNA nanogels, was found to induce antigen-specific antibodies (Rattanakiat et al. 2012; Nishikawa et al. 2014). Thus, these DNA nanoparticles or nanogels composed of 50–100 μg OVA and 100–2,200 μg CpG-containing DNA potently induced T-cell-dependent antibody responses (Rattanakiat et al. 2012; Nishikawa et al. 2014).
Given the current understanding of DNA sensing, attention should be focused toward minimizing autoreactivity of self-DNA molecules. Due to the programmability of DNA nanostructures, improved design of more stable complexes of DNA-based vaccines may afford good immunogenicity with a small amount of antigens. To test this hypothesis, we took advantage of the three-dimensional DNA-tetrahedron structure as our vaccine platform, a design initially developed by Mao’s group (Zhang et al. 2012). This structure has several unique features that enable rational assembly of the vaccine: (1) simple assembly from four DNA strands, (2) well-defined particulate structures with 15–20 nm in size, (3) sufficient lattice for antigen/adjuvant attachment, and (4) presence of streptavidin (SA) that not only stabilizes three-dimensional DNA nanostructures but also serves as a model antigen for eliciting T-cell-dependent humoral responses.
We tested the immunogenicity of SA-specific antibody responses since SA antigens were assembled within DNA nanostructures, as illustrated in Fig. 15.2a. Immunogenicity of these vaccines was examined under a low dosage of antigens and CpG (5.5 μg and 2 μg CpG administered per mouse) (Liu et al. 2012). As a control, the mice were immunized with a mixture comprised of the same amount of antigen and adjuvant. As shown in Fig. 15.2b, the SA linked to DNA tetrahedrons engineered with CpG molecules is highly immunogenic, generating strong and long-lasting anti-SA antibody responses, much more potent than the one induced by SA mixed with CpG. Meanwhile, none of the immunized mice showed any anti-DNA reactivity (Liu et al. 2012). Thus, DNA tetrahedron presents a good platform to induce effective antibody responses. Furthermore, compared to other nanomaterials, the tunable DNA nanostructure offers more structural complexity and flexibility for modifications in order to further improve immunogenicity of the antigen.
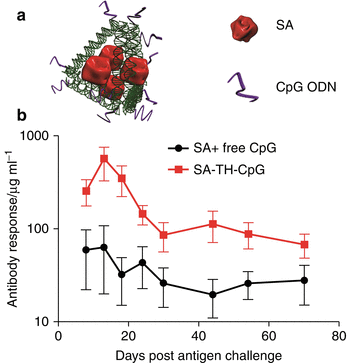
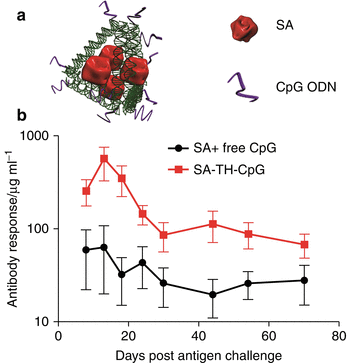
Fig. 15.2
DNA-scaffolded streptavidin (SA) vaccine and its antibody response in BALB/C mice. (a) Cartoon of the tetrahedron DNA-scaffolded vaccine complex. The antigen, SA, and adjuvant, CpG ODN, are assembled onto the same DNA-tetrahedron nanostructure to form the vaccine complex. (b) Anti-SA IgG antibody level in mice after challenge with free SA protein. Mice were immunized twice with different vaccines and challenged with the same free SA. The averages are calculated from at least eight mice, and the error bar represents standard deviation (Reprinted with permission from (Liu et al. 2012). Copyright (2012) American Chemical Society)
15.5 DNA-Tetrahedron-SA Platform for Self-Assembly of Nicotine Vaccine Particles
Given the promising response elicited by DNA-tetrahedron-SA, we decided to extend this vaccine platform to the design and construction of nicotine vaccines. The well-characterized structural information in both SA and DNA tetrahedron makes it possible to engineer vaccine complexes with predefined valences and configuration of nicotine epitopes and CpG molecules.
To create vaccines that allow co-delivery of antigen and adjuvants, two forms of CpG-containing nicotine vaccines were constructed, a soluble antigen, nicotine-SA conjugate linked to biotinylated CpG (Nic-SA-CpG), and a DNA-scaffolded nanoparticle 15–20 nm in diameter. The same nicotine-SA conjugate was attached to a DNA tetrahedron that was self-assembled from biotinylated DNA strands, CpG-containing oligonucleotides, and other strands, leading to the same antigen to adjuvant ratio as that of nicotine-SA-CpG conjugate, as depicted in Fig. 15.3a. Thus, these two types of nicotine vaccines have identical hapten-protein conjugates and the same stoichiometry of antigen and CpG. Their immunogenicity can be directly compared. In addition, given the recently reported finding of an innate stimulatory effect of particulate antigens during the priming, but not boosting process (Link et al. 2012), we inquired whether priming with our particulate nicotine vaccines followed by boosting with soluble Nic-SA-CpG conjugates would elicit stronger nicotine-specific antibody responses. Although soluble Nic-SA-CpG conjugates gave rise to a potent anti-nicotine response (Fig. 15.3b), the priming with DNA-based vaccines followed by challenging with Nic-SA-CpG conjugates rendered a much more durable response as the antibody titer remains elevated 10-week post-third immunization and even surpasses the level of our positive control, nicotine-KLH (Nic-KLH) conjugates mixed with CpG (Fig. 15.3c). The high antibody titers resulted in significant sequestration of nicotine within the serum and blocked the nicotine from entering into the brain. Upon further analysis of the quality of antibody responses, the quantity and affinity of nicotine-specific antibodies were found to be higher in the prime-boost group than in the group immunized with Nic-SA-CpG conjugates and the positive control (although there is no statistically significant difference among these groups). Nevertheless, the promising trend of this immunization regime validates the feasibility of our DNA-based vaccines as a priming agent to induce a high-quality nicotine-specific antibody response. More importantly, the well-defined composition and structural information of soluble and particulate vaccine formulations and the flexibility of combining the two for priming and boosting open opportunities for further optimization of nicotine vaccines and immunization regimes. Finally, the application of DNA-based vaccines as priming agents reduces the chances of adverse autoimmune effects resulting from overt activation in response to synthetic DNA scaffolds.
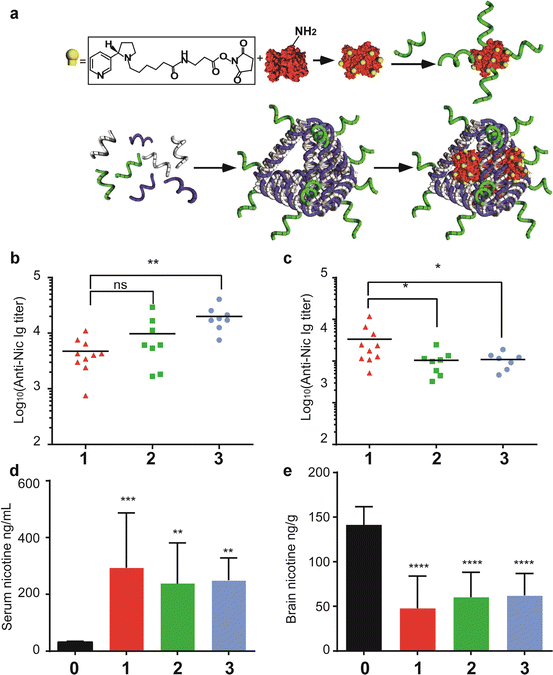
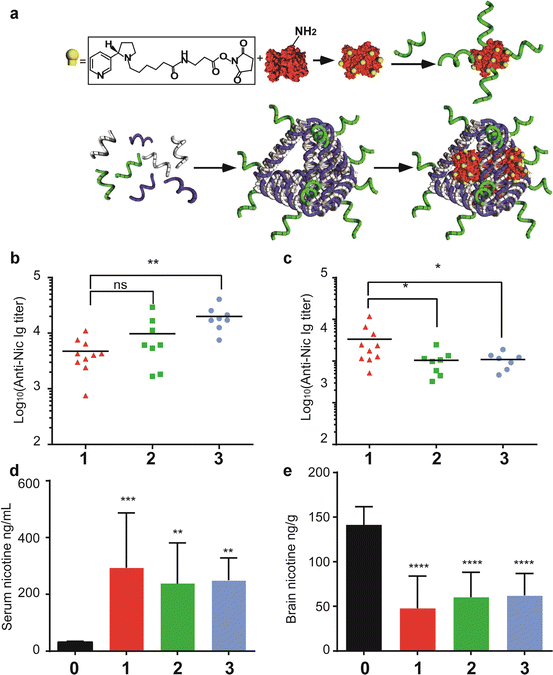
Fig. 15.3
DNA-scaffolded nicotine vaccines, structural features, immunogenicity, and pharmacokinetics. (a) Nicotine hapten (sphere-cylinder symbol) is conjugated to streptavidin (SA) through reaction between NHS ester and primary amine. The conjugated form, Nic-SA, was linked to biotinylated CpG ODN (helical symbol) to form Nic-SA-CpG conjugates. Tetrahedron DNA (TH) is assembled from its component strands, including that containing CpG, through base pairing, and Nic-SA was loaded to the assembled DNA product, TH-CpG, via biotin binding, thereby forming a Nic-SA-TH-CpG vaccine complex. (b, c) Anti-Nic antibody level after the third immunization. (b) 9-day post-third immunization; (c) 78-day post-third immunization. Group 1, prime-boost group; group 2, direct linkage group; group 3, KLH/Nic mixed with CpG group. * represents p < 0.05 compared to prime-boost group, and ** represents p < 0.01, as measured by t-test with Welch’s correction. (d, e) pharmacokinetic analysis of s.c. injected nicotine distribution in mouse serum and brain. (d) shows serum nicotine concentration and (e) shows brain nicotine concentration. Group 0 represents naïve mice without immunization. ** represents p < 0.01, and **** represents p < 0.0001 compared to naïve mice, all as measured by one-way ANOVA with Dunnett’s correction
DNA nanostructures are feasible as a platform for construction of vaccines against nicotine, a small molecule hapten. Certainly, further experimentation is needed to improve nicotine immunogenicity. According to recent understanding of B-cell responses to particulate antigens, various parameters, including particle size, display and density of antigenic epitopes, and attachment of adjuvant molecules, should be considered in future design of effective B-cell vaccines. Given their robust assembly, precision control, and facile introduction of functional groups, tunable DNA nanostructures offer a versatile platform for modular optimizations on all these parameters. In particular, we are focusing on the following tasks: (1) modifying the size and stability of DNA nanoparticles to enhance particle delivery to FDC to promote direct interaction with nicotine-specific B cells; (2) increasing epitope density by incorporating nicotine to DNA molecules at predefined positions and valences for better BCR cross-linking; and (3) optimizing the number and presentation of CpG moiety to enhance adjuvant activity. Furthermore, our finding of DNA-based vaccines as a promising agent in priming simplifies the screening of various DNA nanostructures on the basis of their immunogenicity. Informed application of programmable DNA nanostructures empowers the development of optimized nicotine vaccines.
References
Ahn J et al. STING manifests self DNA-dependent inflammatory disease. Proc Natl Acad Sci U S A. 2012;109(47):19386–91.PubMedCentralPubMedCrossRef