Fig. 12.1
Stimuli of SR polymers
The responses are manifested as variations in the shape, surface, solubility, degree of intermolecular association and others [17]. Particularly, the subsequent polymer structure and property alterations lead to the overall characteristic switching. The extraordinariness of these polymers lies not only in the fast structural macroscopic changes but also these transitions being reversible. Therefore, the polymer is capable of returning to its initial state as soon as the trigger is removed [13, 14]. There is a great deal of literature available about the different types of SR polymers. These materials have been utilized in the form of cross-linked hydrogels, reversible hydrogels, micelles, modified interfaces and conjugated solutions.
The main aim of this review is to highlight the various smart polymeric materials and the drug delivery systems (DDS) that exploit them. These are useful to realize biomimetic devices [18], intelligent medical instruments and auxiliaries [19, 20], electrochemical devices [21], artificial muscles [22], heat shrinkable materials for electronics packaging [23], microelectromechanical systems [24] and actuators and sensors [25].
Stimuli-Responsive Polymers
The stimuli that commonly drive the changes within SR polymers are represented in Fig. 12.1 [5–7]. These ones, commonly classified as physical, chemical, or biological, are also classified as both external or internal stimuli. The polymeric SR materials are too known as self-regulated devices, where the release rate is controlled by a mechanism of feedback that is produced within the body to control the structural changes in the polymer network and exhibit the desired drug release, without any external mediation [26, 27]. On the other hand, externally controlled SR polymers depend on the applied stimuli that are produced with the support of different stimuli-generating strategies, which results in pulsed drug delivery which may be defined as the rapid and transient release of a certain amount of drug within a short time period immediately after a predetermined off-release period [15, 26, 27].
Physically Dependent Stimuli
A very effective way of achieving site-specific drug targeting is by employing externally regulated polymeric drug delivery systems that are responsive to stimuli such as temperature, electric field, light, ultrasound, magnetic fields and mechanical deformation that typically modify the polymeric chain dynamics. Temperature is the most common stimulus for SR polymers.
Temperature-Responsive Polymers
Temperature-sensitive or thermo-responsive hydrogels and polymers have attracted great attention in different fields because some diseases manifest a temperature modification [28, 29]. In particular, they are characterized by a critical solution temperature (CST) around which the hydrophobic and hydrophilic interactions between the polymeric chains and the aqueous media brusquely change within a small temperature range bringing to the disruption of intra- and inter-molecular interactions and resulting in chain collapse or expansion. Typically, these polymer solutions possess a temperature above which one polymer phase exists, namely upper critical solution temperature (UCST), and below which a phase separation occurs with the formation of an hydrogel [30]. On the other hand, polymer solutions that appear as monophasic below a specific temperature and biphasic above it generally possess a so-called lower critical solution temperature (LCST). The LCST is a fascinating phenomenon found for various water soluble polymers which tend to phase-separate from solution upon heating. The most investigated temperature-responsive polymer featuring a LCST in water is the poly(N-isopropylacrylamide) (PNIPAAm). The LCST of PNIPAAm was of ~32 °C, proximate to the human body temperature. By altering the temperature of PNIPAAm solution in water, its solubility behavior can be reversibly changed from hydrophilic-soluble to hydrophobic-insoluble and thus can be induced externally (Fig. 12.2). Then, above the LCST, the polymer become increasingly insoluble leading to gel formation.
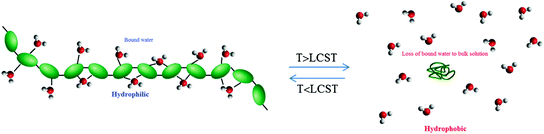
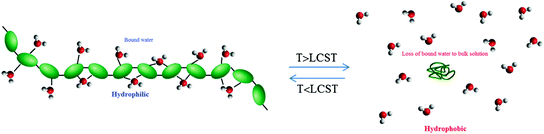
Fig. 12.2
Thermoresponsive polymers: reversible switch from a hydrophilic swollen state to a hydrophobic collapsed state
The phenomenon of transition from a solution to a gel is commonly referred to as sol-gel transition. The sol–gel transition of thermosensitive hydrogels can be experimentally verified by a number of techniques such as spectroscopy [31–33], differential scanning calorimetry (DSC) [31, 32] and rheology [33].
Other N-substituted polyacrylamides [34, 35], and further classes of polymers such as poly(oligoethyleneoxide-(meth)acrylate)s [36], poly(2-oxazoline)s [37], poly(N-vinylalkylamides), e.g. poly(N-vinylcaprolactam) (PNVC) [38], copolymers such as poly(L-lactic acid)-poly(ethylene glycol)-poly(L-lactic acid) (PLLA-PEG-PLLA) triblock copolymers [39], poly(ethylene oxide)-poly(propylene oxide)-poly(ethylene oxide) (PEO–PPO–PEO) copolymers [40] and many natural polymers [41], e.g. polysaccharides and proteins, utilize temperature change as the trigger that determines their gelling behavior without any additional external factor. Researchers have used them alone or in combination to fabricate thermally responsive hydrogels with desired properties. Hydrogels based on these polymers have been interesting for biomedical uses as they can swell in situ under physiological conditions and provide the advantage of convenient administration.
Temperature-Responsive Polymers Applications
The major advantage of thermosensitive polymeric systems is the avoidance of toxic organic solvents, the ability to transport both hydrophilic and lipophilic drugs, reduced systemic side effects, site-specific drug delivery, and sustained release properties.
Hydrogels responsive to temperature are being used to obtain an on–off drug release profile in response to a gradual change of temperature [42–44]. In particular, hydrogels PNIPAAm-BMA based loading indomethacin were analyzed for their on-off release profile. The on state was reached at low temperatures and off state at high temperatures. This behavior is due to the formation of scarcer permeable gel surface layer when the temperature was rapidly changed. This layer acts as barrier whose formation was regulated through the length of the methacrylate alkyl side-chain [45]. Thermo-responsive polymers were used also to overcome the limited therapeutic activity and insolubility of antitumoral drugs due to their toxicity and to the limited accessibility of tumors. The literature reports on the development of different systems (prodrugs, liposomes, micro- and nano-particles), such as anti-cancer drugs carriers, whose therapeutic efficacy is rather limited [46, 47]. Instead, soluble polymeric drug carriers have proved capable to increase drug tumor permeability [48] but, these carriers fail to intrinsically target a specific physiological compartment.
Thermo-responsive polymeric micelles can be used to target adriamycin at the tumoral site. In particular, block copolymers containing hydrophobic polymers, such as poly(butyl methacrylate) (PBMA) and end-functionalized PNIPAAm [49–51], forming a micellar structure in aqueous solution below the transition temperature of PNIPAAm, act as an inert material in the hydrated form. Upon 32 °C, the polymeric chains became hydrophobic due to their dehydration and aggregation and precipitation occur. The cores of micelles then acted as a reservoir for the hydrophobic drug adriamycin.
However, use of PNIPAAm is limited due to cytotoxicity attributed to the presence of quaternary ammonium in its structure, its non-biodegradability and its ability to activate platelets upon contact with body fluids. Many efforts have been made to decrease the initial burst drug release related to thermosensitive systems due to slow in vivo sol–gel transition. Literature data suggest that significant progress in release characteristics can be achieved by optimizing the chain-length ratio between hydrophilic and hydrophobic segments. A novel triblock polymeric system poly(ε-caprolactone)-block-poly(ethylene glycol)-block-poly(ε-caprolactone) (PCL-PEG-PCL) showed a noticeable decrease in initial burst release by coupling to a peptide. Moreover, in vitro drug release studies showed a wide-ranging sustained-release profile for over 1 month [52] (Fig. 12.3).
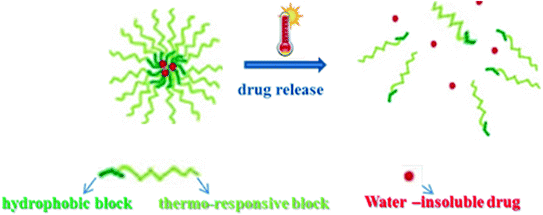
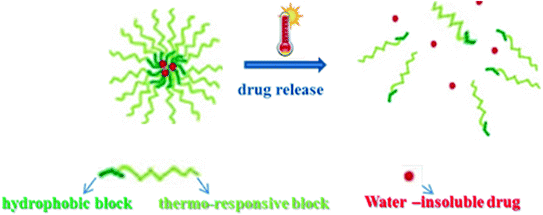
Fig. 12.3
Drug release from a polymeric system
With the aim to develop a drug delivery system with thermal stimuli responsing, Nozawa et al. have been investigated liquid crystal (LC)-entrapped membranes, polymer alloyed membranes and LC-adsorbed membranes for the transport and release of indomethacin. Polymer alloyed membranes were obtained by polymerizing acrylic monomers in presence of LC and LC-adsorbed membrane were obtained by adsorbing LC into porous hydrophobic polymer membrane. Permeation experiments showed that below and above the gel-liquid crystal phase transition temperature of the LC, the extent of thermo-sensitivity for LC-adsorbed membranes was greater than that for the alloyed membrane. The permeability ratio was found to be about 120 with the LC-adsorbed membrane. The obtained results indicated that the LC-adsorbed membrane was an useful candidate as a thermo-responsive system for DDS [53].
Photo-Responsive Polymers
Photo-sensitive polymers have received increasingly attention in recent years because light can be applied straightaway and under specific conditions with high accuracy. The light is both negligibly absorbed by both cells and tissue and greatly consequently by the polymers. This makes light-responsive polymers highly advantageous for applications in wide fields including drug release, biomaterials, and artificial tissues [54–60]. Photo-responsive polymers contain light-sensitive chromophore moieties. Without external stimuli, these polymers can maintain their structures but, upon light absorption, these moieties can be broken from the polymer chain that can be degraded into smaller molecular fragments. Generally, light-sensitive polymers, UV or visible light sensitive, are classified into two main categories that are the side-chain-type polymer containing the chromophores as lateral groups of the chain backbone and the main-chain-type structure possessing single or multiple photosensitive species covalently- or non-covalently connected to the backbone. Chromophores groups are azobenzene [11, 61], spiropyran [62, 63] and nitrobenzyl [64, 65] and a variety of azobenzene or spiropyran-containing photo-responsive polymers such as poly(acrylamide) (PAA) [41, 66] and PNIPAAm [43, 44].
Photo-Responsive Polymer for the Drug Controlled Delivery
UV-sensitive hydrogels could be synthesized by introduction of bis(4-dimethylamino) phenylmethyl leucocyanide, a leuco derivative molecule, into the polymeric matrix in which ionization of the leuco derivative with UV radiation result in discontinuous swelling caused by an increase in osmotic pressure within the gel due to the appearance of cyanide ions formed by UV irradiation [67]. When the UV light is removed the hydrogels shrink. Instead, visible light–responsive materials can be prepared [68] by introducing trisodium salt of copper chlorophyllin, a light-sensitive chromophore, to PNIPAAm hydrogels. When the light is applied the chromophore absorbs it which is dissipated as heat giving an increase of the hydrogel temperature that alters its swelling promoting the drug release.
Polymeric vesicles obtained from the self-assembly of a photocleavable amphiphilic block copolymer, as a light-triggered DDS, were also investigated. The vesicles disintegrate upon UV irradiation, yielding small micellar-like structures, and simultaneously releasing their payload. The versatility of these system was tested both for low molecular weight molecules, proteins, enzymes and DNA. By varying the UV intensity, the loaded drug was released in a controlled manner [69].
Recently, spiropyran-initiated hyperbranched polyglycerol (SP-hb-PG) micelles were reported [70]. These carriers responded to UV/visible light and could dissociate due to conversion of the hydrophobic chromophore SP to zwitterionic and hydrophilic merocyanine (ME). In addition, chromophores such as coumarin, o-nitrobenzyl, stilbene, dithienylethene and 2-diazo-1,2-napthoquinone (DNQ) have been employed in light-responsive micelles, which can respond either to UV/visible or NIR irradiation to undergo structural or phase changes and trigger the drug release from micelles [71–74].
Ichimura and coworkers reported about photo-responsive polymer membranes based on poly(vinyl alcohol) derivatives having AZB side chains with different lengths, filled with liquid crystal (LC), and investigated the photo-response of LC alignment. They found that liquid crystal alignment changes were possible if the AZB unit was linked to poly(vinyl alcohol) backbone by a sufficient long spacer. The response times could be reduced by using high intensity sources [75]. Moreover, when visible light illuminates the surface of the membrane, the azobenzene moieties straighten out and the liquid crystals fall into line, which allows drug to easily flow through the holes. But when ultraviolet light illuminates the surface, the dye molecules bend into a new shape and the liquid crystals scatter into random orientations, clogging the tunnel and blocking drug from penetrating (Fig. 12.4).
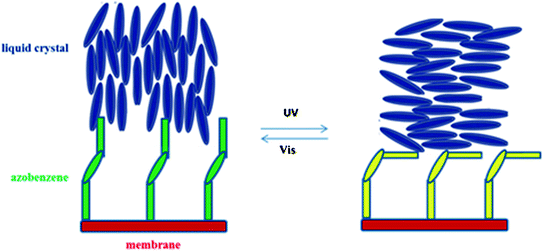
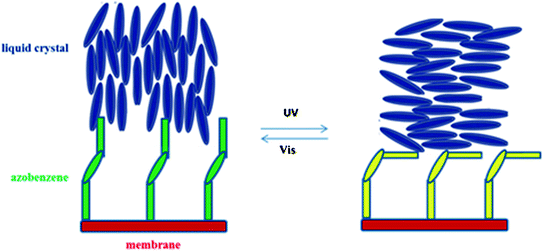
Fig. 12.4
Photo-controlled alignment in liquid crystals
Electro-Responsive Polymers
Increasing research and development efforts have been dedicated to the field of electro-responsive polymers (ERPs) due to their advantages of precise control via the magnitude of the current, the duration of an electrical pulse or the interval between pulses [76, 77]. ERPs are promising candidate materials for the design of drug delivery technologies, especially in conditions where an “on‐off” drug release mechanism is required. Typical ERPs are naturally occurring polymers such as chitosan, alginate and hyalouronic acid are commonly employed to prepare electro-responsive materials. Major synthetic polymers that have been used include polythiophene (PT) or sulphonated-polystyrene (PSS), polyaniline, polypyrrole, ethylene vinyl acetate, and polyethylene.
In some cases, combinations of natural and synthetic polymers have been used. Most polymers that exhibit electro-sensitive behavior are polyelectrolytes and they undergo deformation under an electric field due to anisotropic swelling or de-swelling as the charged ions move towards the cathode or anode (Fig. 12.5). Greatest stress is felt by the region surrounding the anode and smaller stress near the vicinity of the cathode. This stress gradient contributes to the anisotropic gel deformation under an electric field [78, 79].
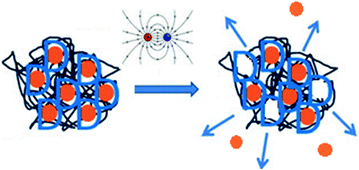
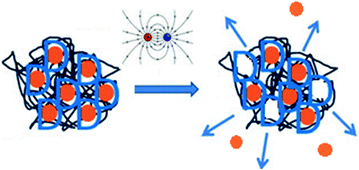
Fig. 12.5
De-swelling of the polymeric drug delivery device due to electric field
Neutral polymers that exhibit electro-sensitive behaviour require the presence of a polarizable component with the ability to respond to the electric field. A rapid bending of gel in silicon oil was observed in the case of lightly cross-linked poly(dimethylsiloxane)-containing electrosensitive colloidal SiO2 particles.
These polymers can show swelling, shrinking or bending in response to an external field [80, 81] and they may be blended into responsive hydrogels in conjunction with the desired drug to obtain a patient‐controlled drug release system useful for neurotransmitters and vaccine delivery. The “on‐off” drug release mechanism can be achieved through the environmental‐responsive nature of the interpenetrating hydrogel‐EAP complex via (i) charged ions initiated diffusion of drug molecules due to an increase in osmotic pressure in the polymer; (ii) conformational changes that occur during redox switching of EAPs; or (iii) electro‐erosion. For example, when an electrochemical stimulus is applied to multilayer polyacrylamide films, the combined effects of H ions migrating to the region of the cathode and the electrostatic attraction between the anode surface and the negatively charged acrylic acid groups lead to shrinking of the film on the anode side [78, 82].
The hydrogel‐EAP composites include other implications such as, application towards biosensors, DNA hybridizations, micro‐surgical tools and miniature bioreactors and may be utilized to their full potential in the form of injectable devices as nanorobots or nano‐biosensors.
Electro-Responsive Polymeric Drug Delivery Systems
ERPs poly(2-acrylamido-2-methyl-propane sulphonic acid-co-n-butylmethacrylate) based were used to delivery edrophonium hydrochloride and hydro-cortisone in a pulsatile manner [83]. The control of drug release was achieved by varying the intensity of electric stimulation in distilled deionized water. For a positively charged drug, the release pattern depends on ion exchange between hydrogen ion produced by electrolysis of water and positively charged solute.
Liu and coworkers have been also designed and successfully realized a novel micro-electro-mechanical-systems (MEMS) based polymer drug delivery microsystem. The device consists of an array of metallic contacts, able to create an uniform electric field. In particular, a hydrogel polymer matrix loaded with hematoxylin dye, as model of hydrophilic drug, has been studied. The delivery microsystem operated at normal body temperature (37 °C) under an applied voltage of 20 V. The release rate and dose were accurately controlled. The polymer responds to the electrical stimulus by shrinking and releases the hematoxylin dye into solution. The release of hematoxylin in the media was monitored using ultraviolet-visible spectrophotometry.
Different drugs can be encapsulated within the hydrogel polymer matrix. The de-swelling of the polymer upon exposure to the applied electric field allows the encapsulated drug to be released from the matrix. The control of the applied voltage can be used to achieve pulsatile drug delivery. Alternatively, small volumes of drug may be continuously delivered to maintain the optimal therapeutic dose for the patient [84].
An electro-responsive drug delivery system was also developed using poly(acrylamide-grafted-xanthan gum) (PAAm-g-XG) hydrogel for transdermal delivery of ketoprofen [85]. When a swollen PAAm-g-XG hydrogel was placed between a pair of electrodes, a de-swelling of the hydrogel was observed in the vicinity of electrodes carrying the electric stimulus. Ketoprofen-loaded PAAm-g-XG hydrogel was also crosslinked with poly(vinyl alcohol) to prepare films as rate controlling membranes (RCM). The in vitro drug permeation study from the formulations was performed through excised rat abdominal skin. The drug permeation across the skin was significantly improved in the presence of electric stimulus as compared to passive diffusion and was found to be dependent upon the applied electric current strength and crosslink density of RCM. A pulsated pattern of drug release was observed as the electric stimulus was switched on and off. These PAAm-g-XG hydrogel could be useful as transdermal drug delivery systems actuated by an electric signal to provide on-demand release of drugs.
Pristine multi-walled carbon nanotubes (pMWNTs) were incorporated into a polymethacrylic acid (PMAA)-based hydrogel matrix by in situ radical polymerisation [86]. The effect of pMWNTs and cross-linker concentration on the electrical and mechanical properties of the hydrogel was carefully studied. The incorporation of pMWNTs into the polymeric network improved the electrical properties of the hydrogel. Moreover, the drug release from the gels was significantly enhanced at high pMWNT concentrations. But, the presence of pMWNTs within the hydrogel matrix affected the hydrogel mechanical properties by decreasing the pore size and, consequently, the swelling/de-swelling of the gels. The damage on the gel surfaces after electrical stimulation and the loss of the pulsatile release profile at high cross-linker concentrations, suggested that the mechanism of drug release involved a compacting effect and intensified the stress on the polymeric network as a result of the electrical properties of pMWNTs.
Ultrasonically Responsive Polymers
An innovative approach of exploiting ultrasound in drug delivery consists on the application of the ultrasonic waves directly at the polymers or the hydrogel matrix [87]. For the ultrasonically responsive polymers the release mechanism is driven by the cavitation. In particular, the ultrasound (ULS) energy generates both high and low pressure waves, resulting in an alternative growth and shrinkage of gas-filled microbubbles. These high-low pressure waves regulate the intermittent opening of the pores of the polymer, thus inducing the delivery of the respective drugs.
The most widely employed polymers for the ULS-responsive uses could be biodegradable or non-biodegradable. The biodegradables ones include polyglycolides, polylactides, bis(p-carboxyphen-oxy)alkane anhydride with sebacic acid. Instead, the non-biodegradables materials are ethylene-vinyl acetate copolymers or the poly(lactide-co-glycolide) microspheres, PHEMA hydrogels, the PEO-b-PPO-b-PEO micelles and the poly(HEMA-co-DMAEMA) hydrogels. The releasing agents are p-nitroaniline, p-amino-hippurate, bovine serum albumin and insulin. When exposed to ultrasound, these bioerodible polymers respond rapidly and reversibly. It is believed that the ultrasound also causes an increase in temperature in the delivery system, which allows the diffusion [88, 89]. ULS-responsive polymers are advantageous because they are non-invasive and capable of penetrating deep into the interior of the body and thus drug delivery can be focused and carefully controlled through a number of parameters including frequency, power density, duty cycles and time of application [90, 91]. Biologically the ultrasound action is related to the generation of thermal energy, perturbation of cell membranes, and enhanced permeability of blood capillaries [92].
Application of Ultrasound in Drug Delivery
Ultrasound responsive drug delivery systems have great potential for applications requiring stimulated release in vivo with a high degree of control over spatial and temporal location. Numerous carriers for ultrasonically enhanced drug delivery have been explored including polymeric ultrasound contrast agents with targeting potential, modified lipospheres and nano-/micro-bubble-enhanced chemotherapy (Fig. 12.6) [93–97]. Miyazaki and coworkers used ULS-responsive matrix ethylene-vinyl acetate copolymer (EVAc) based to achieve a 27-fold increase in the release of 5-fluoruracile. The ultrasound strength increase results in a proportional increase in the amount of 5-FU release [98]. EVAc systems were also tested as delivery device of insulin. It was found that exposure of insulin-loaded matrices to ULS significantly reduced the blood glucose levels on the rats [99].
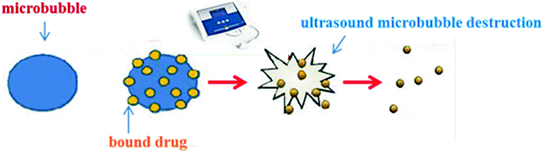
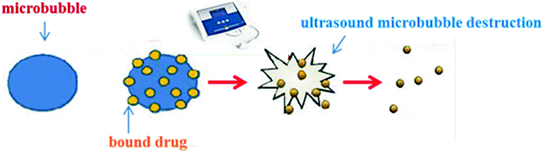
Fig. 12.6
Action of ultrasound targeted microbubble destruction. Microbubbles are attached with drugs or genes
Kwok et al. have reported on the development of ULS-sensitive barrier membranes able to give a pulsatile drug release. A co-polymer of 2-hydroxyl methacrylate (HEMA) and PEG dimethacrylate was loaded with particulate insulin and PEG, after which the surface of the polymer was coated with methylene chains. Upon exposure to ultrasound the methylene chains became disordered, permitting the insulin to diffuse out of the matrix [100, 101].
In addition, Suzuki et al. [102] achieved tumor-specific ultrasound-enhanced gene transfer with novel liposomal bubbles, which entrapped an ultrasound imaging gas. Extraordinarily, the bubble liposomes healthy transferred genes, only at the site of ultrasound exposure, into tumor cells and solid tumor tissue [103–106].
Magnetically-Responsive Polymers
The use of an oscillating magnetic field to modulate the rates of drug delivery from a polymeric matrix was one kind of method to achieve externally controlled drug delivery systems [107]. When planning a magnetic-responsive delivery system characteristics such as the magnetic properties of the carrier particles, field strength and geometry, drug/gene binding capacity and physiological parameters like the depth to target, the rate of blood flow, vascular supply and body weight need to be considered [108]. Magnetic targeting is based on the attraction of magnetic polymer carrier to an external magnetic field source that effectively traps it in the field at the target site and pulls it toward the magnet [109–113]. In order to maintain the magnetic loaded carrier at a specific location, the externally applied field must have a relatively strong gradient. When the drug is released from the magnetic matrix this is no longer responsive to the applied field.
Several carriers for magnetically enhanced drug delivery have been studied including nanoparticles with a magnetic core and a polymer shell and liposomes which have a magnetic core and an artificial liposomal shell are reported [114–119]. These materials may also be embedded in hydrogels which can carry drugs that are released upon heating [110, 120]. To produce highly efficient magnetic-responsive materials, the “doping” of polymer materials with magnetic nanoparticles (MNPs), made of inorganic matter (most often superparamagnetic iron oxide (SPION) Fe3O4 or g-Fe2O3, or ‘‘soft’’ metallic iron, but also “hard” magnetic materials e.g. Co, Ni, FeN, FePt, FePd…), appeared to be the more appealing and efficient solution.
Many hydrophilic polymers were proposed to host magnetic nanoparticles, in particular thermosensitive gels like poly(N-isopropylacrylamide) (PNIPAAm). However, PNIPAAm and other polymers exhibiting a LCST are generally less polar than polyvinyl acetate (PVA) and can be inefficient for trapping the MNPs due to the absence of hydrogen bonds and to a mesh size of typically 10–20 nm, i.e. larger than the size of superparamagnetic iron oxide MNPs (5–10 nm) [121]. To overcome this problems, strategies reported in the Fig. 12.7 concerning on a statistical copolymer network with a chelating comonomer such as 2-acetoacetoxyethylmethacrylate (AEMA) [122], a semi-interpenetrated network with alginate chains wrapping the MNPs [123] or a composite network of PNIPAAm and poly(ethylene glycol) (PEG) using PEG400–dimethacrylate as crosslinker of NIPAAm [124] were proposed.
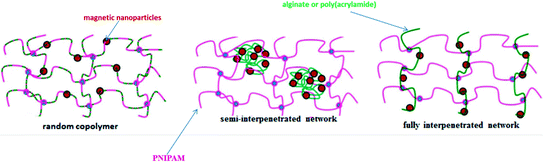
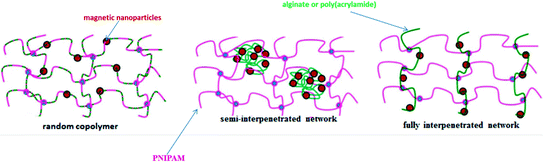
Fig. 12.7
Strategies proposed to trap magnetic nanoparticles inside a hydrogel network
Magnetic Field Responsive DDS
By merging magnetic and polymer materials one can obtain composites with exceptional magnetic responsive features. In this context, magnetic responsive micro- and nano-particles have been explored as possible drug carriers for site-specific drug targeting [125, 126]. Cytotoxic anti-cancer drugs were attached to these carriers and injected into the subject both via intravenous or intra-arterial injection. External magnetic fields generated by rare earth permanent magnets were then used to direct and concentrate the drug at the tumor site [127]. Subcutaneous implants of EVAc-insulin, capable of decreasing glucose levels in diabetic rats for 105 days, were also designed [128, 129]. Additionally, Hsieh et al. embedded magnetic steel beads in an EVAc copolymer matrix that was loaded with bovine serum albumin as a model of drug. They demonstrated increased rates of drug release in the presence of an oscillating magnetic field [117, 130]. During the exposure to the magnetic field, the beads oscillate within the matrix, on the other hand creating compressive and tensile forces. This consecutively acts as a pump to push an increased amount of the drug out of the polymer matrix. In vivo studies were later conducted demonstrating the effectiveness of an optimized version of this system, consisting of EVAc-protein matrices containing magnetic beads, loaded with insulin. In particular, was demonstrated that glucose levels can be repeatedly decreased on demand by applying an oscillating magnetic field [131–134].
Chemically Dependent Stimuli
Stimuli that occur internally are classified as chemical or biological. The pH as well as the ionic strength, redox and solvent are defined chemically-dependent stimuli.
pH-Responsive Polymers
pH sensitive polymers are materials which respond to the changes in the pH of the surrounding medium by varying their dimensions. Such materials increase its size (swell) or collapse depending on the pH of their environment (Fig. 12.8). This behavior is due to the presence of certain functional groups in the polymer chain such as acidic or basic groups that can either accept or release a proton in response to changes in environmental pH.
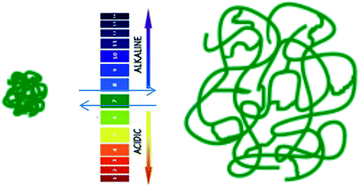
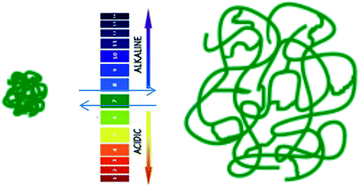
Fig. 12.8
Swelling-de-swelling transition of a pH responsive hydrogel
The pH is an important environmental parameter for biomedical applications, because pH changes occur in several specific or pathological sites.
The changes along the gastrointestinal tract from acidic in the stomach (pH = 2) to basic in the intestine (pH = 5–8) has to be considered for oral delivery of any kind of drug. Certain cancers as well inflamed or wound tissue exhibit a pH different from 7.4 as it is in circulation. For example chronic 7.4 and 5.4 and cancer tissue is also reported to be acidic extracellularly [135–137].
Consequently, this parameter can be exploited for a direct response at a certain tissue or in a cellular compartment. As mentioned above, pH responsive polymers consist of polymers with a large number of ionisable groups known as polyelectrolytes. Polyelectrolytes are classified into two types: weak polyacids and weak polybases. Weak polyacids accept protons at low pH and release protons at neutral and high pH [138]. Poly(acrylicacid)(PAAc) and poly(methacrylicacid) (PMAA) are commonly used pH-responsive polyacids [139, 140]. As the environmental pH changes, the pendant acidic group undergoes ionization at specific pH called as pKa. This rapid change in the charge of the attached group causes alteration in the molecular structure of the polymeric chain. This transition to expanded state is mediated by the osmotic pressure exerted by various stimuli responsible for controlling drug release from smart polymeric delivery systems [26–143]. pH responsive polymers typically include also chitosan [144], albumin [145], gelatin [146], poly(acrylic acid) (PAAc)/chitosan IPN [147], poly(methacrylic acid-g-ethylene glycol) [P(MAA-g-EG)] [148, 149], poly(ethylene imine) (PEI) [150], poly(N,N-diakylamino ethylmethacrylates) (PDAAEMA), and poly(lysine) (PL) [151, 152].
pH-Stimuli Sensitive Polymer DDS
Oral pH sensitive drug delivery systems are gaining importance because they are able to deliver the drug at specific part of the gastrointestine. Antibiotics, especially macrolide ones like erythromycin, enzymes and proteins are rapidly degraded by gastric juices. Others, such as acidic drugs like NSAID’s (e.g., diclofenac, valproic acid, or acetylsalicylic acid) cause a local irritation of the stomach mucosa.
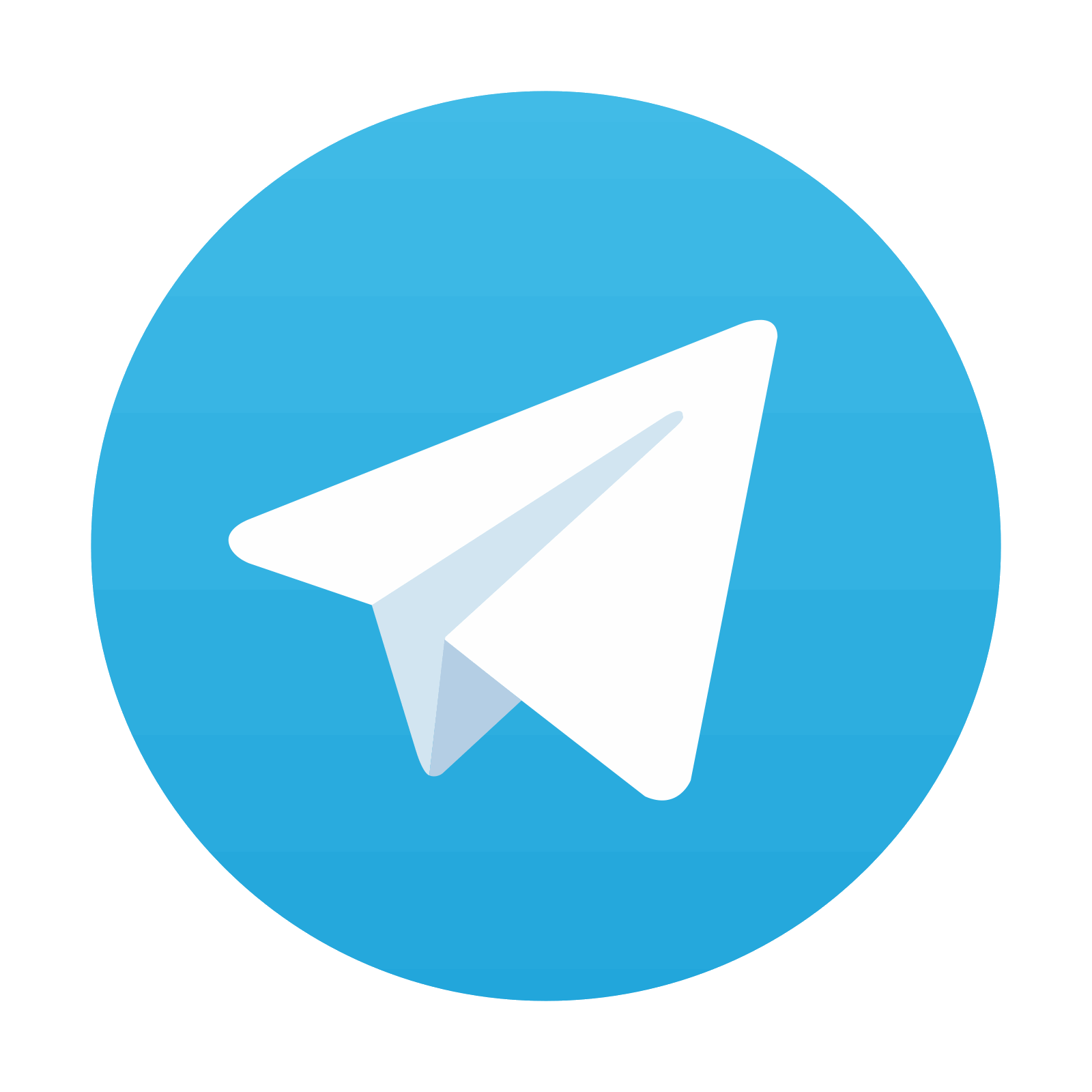
Stay updated, free articles. Join our Telegram channel
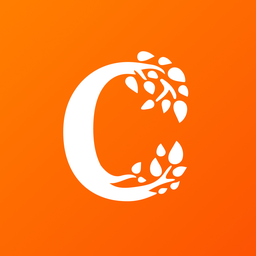
Full access? Get Clinical Tree
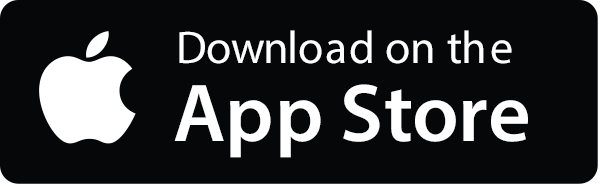
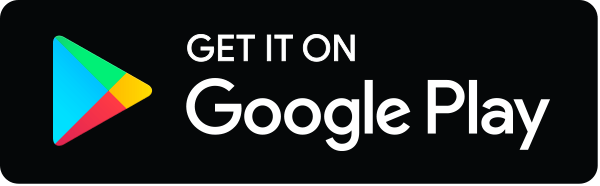
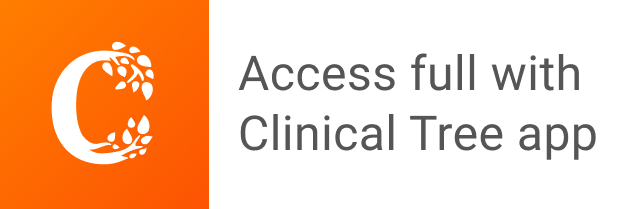