2 Jeffery B. Bylund1 and Antonis K. Hatzopoulos2 1Department of Medicine and Department of Pharmacology,Vanderbilt University, USA 2Department of Medicine, Vanderbilt University, USA Generating cardiac tissue ex vivo to replace damaged or failing myocardium has been a goal of researchers for decades. Recent advances in stem-cell biology allowing the isolation and culture of pluripotent stem cells have made this aspiration a possibility. The complexity of the processes that drive stem cells from a pluripotent state toward progenitor and then specialized cell populations has made in vitro derivation of cardiomyocytes a challenging pursuit. However, a better understanding of the processes involved in cardiac tissue formation on a cellular and molecular level has unlocked the mechanisms that transform a pool of cells in the mid-portion of the embryo into a beating tube and finally a highly efficient, four-chambered double pump. Advances in our understanding of and ability to culture pluripotent stem cells have provided a model system to which to apply our more recent understanding of cardiac developmental mechanisms in vitro. This application has further refined our understanding of the molecular mechanisms behind the cardiomyogenic process and allowed for the creation of large numbers of functional cardiomyocytes in culture. These cultured cardiomyocytes have applications in the fields of drug testing and toxicology, basic research, and eventually as novel therapeutics. In order to illustrate the complexity and exquisite delicacy of the processes whereby heart tissue is formed, we will begin this chapter in vivo, learning from the embryo how a heart is made. This will include a look at the major morphological features of the developing heart and at the timing of their appearance. We will then provide a summary of the major cellular and molecular events involved in the formation of each important feature. After establishing a biological context for the varied events that take place during cardiogenesis, we will move out of the embryo and into the cell culture dish. In this section we will review the different types of pluripotent stem cell and their basic biology, including how they differ from other types of stem cell and what features make them important for the modeling of cardiac development in vitro. The third section will cover the approaches used to generate cardiomyocytes from pluripotent stem cells. This will include strategies for using biological molecules such as growth factors to modulate signaling pathways in an attempt to mimic key developmental signaling events. This section will also cover the use of small molecules and other approaches to modulating these same key developmental pathways. The final section will discuss the applications for stem cell-derived cardiomyocytes. This will include a look at the use of pluripotent stem cell-derived cardiomyocytes in basic research, drug discovery, tissue engineering, and clinical applications. While our understanding of the anatomical and morphological features of heart development spans more than a century, it is only recently that we have begun to unlock the cellular and molecular mechanisms of this amazingly complex process. In this section we will review how a small number of progenitor cells in the early embryo are able to transform into a four-chambered, life-giving pump. Before any organs begin to form, the developing embryo must transition from a mass of cells into layers of tissue that will eventually form a complete organism. The process by which these primitive layers of tissue form is known as gastrulation. At gastrulation the embryo transforms from a sphere of dividing cells into an organized, multilayered disk (human) or tube (mouse) called a gastrula [1,2]. The position of each layer of tissue generally lends itself to the eventual positioning of the various organs. The skin will form from the topmost outer layer, the ectoderm, while the inner and bottom layers, the endoderm and mesoderm, will form the gut, the muscles, the heart, and other organs. It is after gastrulation that the heart begins to form from the mesodermal layer. In the post-gastrulation embryo the heart is the first organ to form and is the first functional organ, pumping blood and providing nutrients to diffusion-limited cells as the embryo develops. The complete heart is made up of several cell and tissue types. Innermost is the endocardium, a layer of endothelial cells that line the chambers and major vessels coming into and stemming from the base of the heart. In the middle of the wall of the heart is the largest portion by volume and mass, the myocardium. The myocardium consists of cardiomyocytes, which are responsible for the contractile force of the heart and for the propagation of membrane depolarization that drives regular beating. The myocardium is composed of a combination of several types of cardiomyocyte, each of which is functionally and structurally distinct. These distinctions are the result of the functionally distinct role of each region of the myocardium during contraction. The atria are primarily responsible for regulating blood flow into and out of the ventricles, helping to maintain a proper rhythm. The ventricles provide most of the contractile force generated during a heartbeat. Connecting the atria and ventricles are bundles of mostly “nonworking” cardiomyocytes that initiate and propagate the electrical signals which cause the heart to beat. These nonworking cells are generally referred to as conduction system cells. The outermost layer of the heart is the epicardium. This is a layer of connective tissue that serves as a covering and provides mainly smooth muscle cells to support the coronary arteries. During development, each of these layers must form at the right time and in the right place in order to provide a fully formed and functionally normal heart. While the heart is not fully grown until after birth, achieving a mostly complete heart takes a remarkably short amount of time in lower mammals. In the well-studied mouse embryo the progression from progenitor cells to a functional four-chambered heart takes only about a week [3]. Arriving at this final configuration requires passage through what may be described as four major stages: specification of early cardiac progenitors and their coalescence into cardiogenic regions called heart fields; fusion of these cells at the midline to form the heart tube; cardiac looping and primitive chamber formation; and finally cardiac remodeling, including septation and valve formation. The first of these four stages of heart development is the specification and migration of cardiac progenitor cells from the mesoderm of the gastrula. These early cardiogenic regions appear around embryonic day 6.5 in mice, at Hamburger Hamilton stage 3 in chicks and about 22 days postfertilization in humans [4,5]. At the earliest stages, cardiac progenitors are not easily distinguished from other cells in the mesoderm. However, by day 7.5 in the mouse these progenitors have migrated into the midline of the embryo to form the first distinguishable cardiac structure, the cardiac crescent [3, 6]. This crescent, shaped like a C with the open end oriented caudally, is located at the cranial portion of the embryo just below the developing head fold and neural tube. At the cardiac crescent stage cardiac progenitor cells reside in two distinct regions, called the primary and secondary heart fields (PHF and SHF, respectively) [6]. The cells in these fields will eventually become most of the heart. Cells in the PHF eventually become the left ventricle and a portion of the atria and the right ventricle. The SHF is located lateral to the PHF and contributes to the rest of the atria and right ventricle, as well as the entire outflow tract. In addition to their spatial regulation, the cells of the PHF and SHF are also temporally regulated. The cells derived from the PHF are the first to differentiate into cardiomyocytes and are known as first lineage cells. The SHF cells remain in an undifferentiated state until after the PHF cells begin to form the heart tube. During tube formation and throughout looping, SHF cells continue to differentiate and make contributions to the atria, ventricles, and outflow tract [7]. The second stage of heart development is cardiac tube formation. This process starts around embryonic day 8 in mice and is indicated morphologically as a budlike structure emerges at the cranial portion in the PHF of the cardiac crescent [3]. This budlike structure then enlarges as the crescent begins to fuse at the midline, gaining volume while expanding cranially and caudally [8]. At the caudal end of the crescent PHF, cells migrate downward in a bisymmetric fashion on the right and left sides of the emerging heart, forming an inverted Y. At the top of this inverted Y the budding PHF cells, with contributions from the SHF, expand downward to form the heart tube. This tube is a cylindrical structure composed of developing myocardium on the outside and a layer of endothelium called endocardium on the interior. While the PHF is expanding to form the anterior portions of the emerging heart, the SHF is also expanding cranially and posterior to the PHF. The expanding SHF cells make some contribution to the mostly PHF-derived heart tube cells but also form a distinct structure at the top of the inverted Y that will eventually become the outflow tract. The cranial and caudal portions of this tube are known respectively as the arterial and venous poles. Once the heart tube is completely fused between the correctly oriented arterial and venous poles, it begins to act as a rudimentary pump. This pumping action is essential for moving blood through the developing circulatory system of the embryo, which at this point requires blood flow to provide nutrients and oxygen to diffusion-limited cellular compartments. It is also evident that myocardial cells capable of producing contractions are present even in this rudimentary heart. Though the heart is beginning to fulfill its functional role as a pump to move blood through the embryo, it must still detach itself from the mediastinum and orient itself properly for chamber and valve formation. This reorientation process occurs during the third major stage in heart development, cardiac looping. In mice this looping process begins around embryonic day 9.0 and in humans around 5 weeks postfertilization. In mice it takes about 1–2 days to complete. As already described, the newly formed heart tube is at this point an asymmetric structure consisting of a bifurcated region at the venous pole and a straight tube with rudimentary SHF-derived outflow tract at the arterial pole. The bifurcated caudal end contains the inflow tract and what will become the atria. During looping, this pole of the heart moves dorsally and cranially while twisting to the right, bringing it to a cranial position just above the developing ventricles. While the venous pole is reorienting as described, the arterial pole containing the outflow tract is also moving, albeit caudally and ventrally in an opposite and complementary fashion. These movements have now oriented the two poles in an asymmetric dorsal–ventral configuration with the outflow tract on the right and a common atrium and inflow tract on the left. These two regions are connected by a loop of bulbous, asymmetric cardiac tissue that includes the atrioventricular (AV) canal. This bulbous loop will eventually become the left and right ventricles. The former poles will become the atria and major vessels located at the base of the heart. It is during this period of development that the ventricles begin to be separated via the interventricular septum and that the precursors to the valves, or endocardial cushions, begin to form [3, 8]. Now that the heart is positioned properly, it is ready to proceed to the final step of development, cardiac remodeling and definitive formation of the cardiac chambers. As indicated earlier, primitive chambers are already aligned and ready to form the two atria and two ventricles characteristic of the adult heart. The atria form as the common atrium expands to form two appendages. While these atrial appendages are forming, the outflow tract is imposed upon their junction by continued spiraling of the heart tube. The outflow tract and atria fuse, producing two distinct atrial chambers. At the same time the inflow region insinuates itself between the bulging ventricular regions on the dorsal side of the heart. The inflow tract then fuses with the cardiac loop, creating separate left and right ventricles. During this stage the valves and septa that separate the chambers from one another are also forming. The endocardial cushions in the AV canal give rise to the tricuspid and mitral valves, while those in the outflow tract form the septum dividing the outflow tract into the aorta and pulmonary artery [3]. These four steps give a sketch of the complexity of the heart-forming process. Indeed, the procession through these four stages is complete by around E14 in the mouse, yet the heart continues to grow and mature through the remaining week or so until birth, and even thereafter. For the purpose of this chapter, however, these four basic steps provide the proper context for our discussion of the cell types that populate the heart during development and the molecular mechanisms that drive them. It is this molecular understanding, gained by careful inspection of the different stages of heart development, that has allowed us to generate cardiomyocytes in vitro from pluripotent stem cells. In order to appreciate how our understanding of the major events in cardiogenesis has been applied to pluripotent stem-cell differentiation in vitro, it is important to describe the major cell types involved in each of the developmental stages of the heart in terms of their origin, movement, and eventual fate. Coordinating the differentiation and movement of cells during the four stages are major developmental signaling pathways. From mesoderm derivation to remodeling and chamber formation, these signaling pathways act to sequentially regulate cardiac transcription-factor networks. These transcription-factor networks regulate important structural and functional genes that eventually characterize the mature cardial cells. Thus the molecular and cellular events that drive cardiogenesis are best thought of in terms of these signaling pathways and the transcription factors under their control. In the context of cardiomyocyte differentiation from pluripotent stem cells, signals and factors regulating the origin and differentiation of early cardiac progenitor cells are most relevant. We will therefore focus most of our attention on the early events of cardiac differentiation. Prior to gastrulation, stem cells from the inner cell mass (ICM) of the blastula form one of the earliest embryonic tissue types, the epiblast. Cells of the epiblast then give rise to the three primary germ layers. They first form the primitive streak and the earliest of the three germ layers, the ectoderm. The cells of the primitive streak then go on to form the endoderm and mesoderm. This transition of embryonic stem cells toward mesodermal tissue may be classified as the first important transition in vivo that guides our understanding of how to generate cardiomyocytes in vitro from pluripotent stem cells. As gastrulation progresses, epiblast cells migrate through the primitive streak and expand outward between the ectoderm and endoderm [9]. Mesodermal cells continue to expand laterally into the developing space between the ectoderm and endoderm, eventually segmenting into four different sections [10]. Developmental pathways directing the specification, migration, and expansion of mesoderm from epiblast through the primitive streak and into its final position between ectoderm and endoderm include Activin/Nodal, Wnt, BMP, and fibroblast growth factor (FGF) [9, 11]. These pathways act in concert to regulate each other – confining proper activation to specific regions by regulating the expression of agonists and antagonists – and to regulate the expression of mesodermal transcription factors. Early induction of mesoderm is associated with the activity of Activin/Nodal signaling. Nodal is expressed in the epiblast, where it activates BMP signaling, which in turn activates Wnt. Later, Nodal and BMP act together to control the expression of Wnt antagonists such as Dkk1 in order to restrict Wnt signaling to the primitive streak, where it directs the formation of mesoderm. During mesoderm induction the Wnt signaling pathway acts as a fulcrum point for the interaction of Nodal and BMP signaling. As mesodermal tissue continues to form, Wnt signaling is detected toward the posterior end of the primitive streak around E6.5 and then expands into the entire primitive streak and much of the mesoderm by E7.5 [12]. Loss of Wnt signaling components, including Wnt ligands, co-receptors, and β-catenin (the nuclear protein mediating Wnt transcriptional control), results in a failure to form mesodermal tissue [13–17]. Wnt signaling affects the formation of mesoderm mainly via the Wnt ligand Wnt3A. Wnt3A regulates the activation of the essential mesodermal transcription factors T-brachyury (T-bry) and Eomes [18]. In fact, Wnt signaling acts directly on T-bry via translocation of β-catenin to the nucleus and activation of gene transcription [19]. These transcription factors play a critical role in cell migration during mesoderm specification and are used as definitive markers of mesodermal tissue [20]. FGF signaling also plays an essential role in the early formation of mesoderm. Loss of FGF ligands such as FGF8 and receptors such as FGFR1 leads to a loss of mesoderm by interfering with cell migration [21–23]. The effect of FGF signaling on mesoderm formation is likely mediated via its regulation of the transcription factors Snail, T-bry, and Tbx6, three genes required for mesoderm migration, specification, and patterning. This gives a brief sketch of the molecular events required for mesoderm formation, providing context for the next section, where we will examine the formation of cardiac progenitors via the transcription factors that drive cardiac progenitors and the signaling pathways that guide them. It is important to note that mesoderm formation and cardiac-progenitor specification are not necessarily mutually exclusive processes. Many important developmental events occur in concert with one another as the heart develops. Examples of this concept will be apparent as we continue our discussion of the cellular and molecular events involved in cardiac development. Cardiac precursors can be detected in the anterior region of the primitive streak during the early stages of mesoderm formation. These precursors are not yet cardiac cells but are a collection of cardiac progenitors that give rise to the earliest cardiac cells. These early cardiac cells are present on either side of the embryonic midline and express the transcription factors Mesp-1 and Mesp-2. Loss of Mesp expression in these cells causes a failure in the migration required for the movement of emerging cardiac progenitor cells [8, 24, 25]. Expression of Mesp-1 in the cardiogenic mesoderm is a master signal that initiates the cardiac transcription-factor signaling network [24]. Mesp-1 expression rapidly induces the expression of a new set of transcription factors involved in cardiogenesis. These include Nkx2-5, Hand2, Myocd, Gata4, Mef2c, Tbx20, FoxH1, Foxc1, SRF, and Foxc2, among others [24]. The mechanism behind this activation lies in the ability of Mesp-1 to bind directly within the regulatory regions of its target genes, including Nkx2-5, Hand2, and Myocd. Myocd in turn activates Mef2c and SRF, which go on to activate the essential cardiac structural genes Myh6, Myl1, Myl2, Myl7, and cardiac troponin 2 (Tnnt2) [26,27]. Each of the transcriptional events in the formation of cardiac progenitors is thereby linked from Mesp-1 expression in the early mesoderm on through expression of cardiac-specific structural and functional components in mature cardiomyocytes. This point is very important to keep in mind: cardiogenesis on a cellular level occurs via the carefully orchestrated, stepwise movement of progenitor cells toward mature cardiac subtypes, directed by cardiac transcription factors. As we will discuss later, it is upon this principle that most protocols for production of de novo cardiomyocytes from pluripotent stem cells depend. This summary illustrates the sequence of transcription-factor events kicked off by Mesp-1 and propagated onward to drive heart formation. We will now discuss where these transcription factors are expressed and the role they play during cardiogenesis. Some are restricted temporally and spatially to a particular period of heart development, while others are expressed throughout. Nkx2-5, Hand1 and 2, Gata4, and Tbx1,5 and 20 are expressed in the PHF. These transcription factors direct the differentiation of cells within the PHF as they populate the heart tube and differentiate during looping morphogenesis [3, 6, 8]. Nkx2-5 is important in atrial–ventricular chamber compartmentalization and the formation of ventricular tissue. Hand1 and 2 play a direct role in the formation of the left and right ventricles, respectively. Gata4 expression is essential for looping morphogenesis, septation of the valves, and formation of ventricular myocardium. At the stage of heart-tube formation, cardiac cells must migrate and fuse at the midline. Here, Gata4 and a companion transcription factor, Foxp4, are essential for proper tube formation, as mutations in these genes lead to a failure of the cells to fuse and form a proper heart tube [3]. The Tbx transcription factors are an important class that play diverse roles in cardiogenesis, including patterning and specification of conduction system cells and sinoatrial nodal cells and distinguishing between atrial and ventricular myocardium. In fact, patterning via the Tbx genes is the major driver for distinguishing between working and nonworking myocardium during the later stages of cardiac development [28]. Tbx transcription factors are also critical to the formation of the outflow and inflow tracts and neuralization of the atrium at later stages of heart development [29,30]. Tbx20 plays an important role in proliferation of myocardial progenitor cells during early myocardial expansion. Mutant embryos lacking Tbx20 expression present a large decrease in the number of terminally differentiated cardiomyocytes [31]. Tbx18 is expressed in the extreme posterior regions of the SHF [6]. Cells that express Tbx18 end up in diverse cardiac locations, from smooth muscle to myocardium and interventricular septum [6]. Isl1 is another essential cardiac transcription factor. Isl1 expression in the SHF plays a role in many cardiac development processes. Mutations that inactivate or knockdown its expression result in major cardiac defects. These include lack of septation in the atrial and ventricular compartments, abnormal atria, and a lack of outflow tract [32]. Isl1, along with Gata4, can directly control the expression of Mef2c by binding a genomic enhancer element upstream of its coding sequence [33]. Incidentally, Isl1 expression has been detected in the PHF as well as the SHF (where it was originally identified), although it does not seem to be required for formation of the PHF lineages [34]. When Isl1 expression is knocked down in transgenic mice, the PHF and its associated derivatives still form as expected [32].
Directed Cardiomyogenesis of Pluripotent Stem Cells
2.1 Introduction
2.2 A Brief Review of Heart Development
2.2.1 Cellular and Morphological Movements
2.2.2 Molecular Events in Heart Development
2.2.2.1 Molecular Events of Mesoderm Derivation
2.2.2.2 Transcription Factors in Cardiac Development
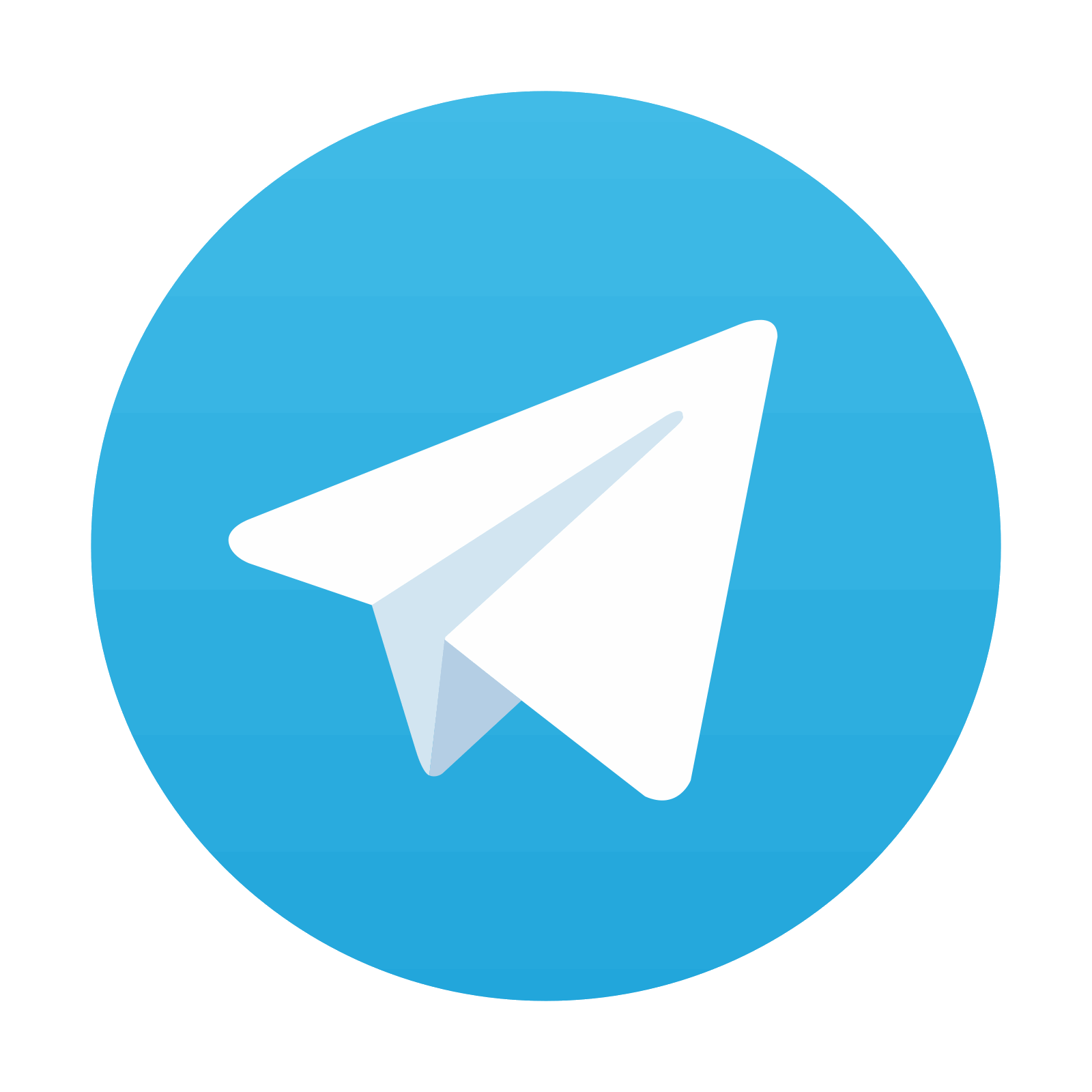
Stay updated, free articles. Join our Telegram channel
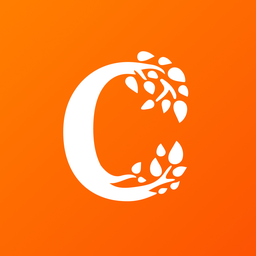
Full access? Get Clinical Tree
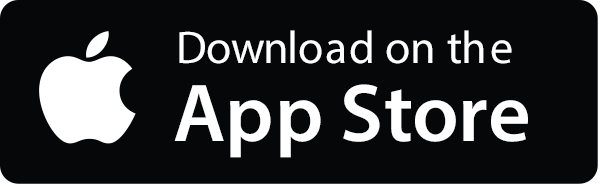
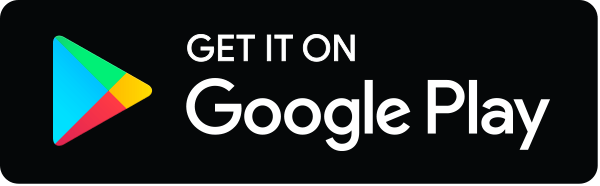