Fig. 1
Principle of single-molecule super-resolution microscopy. (a) Photoswitchable fluorophores are transferred from a fluorescent bright state into a nonfluorescent dark state by the irradiation of light. (b) By activating only a sparse subset of fluorophores at a time, the position of single fluorophores can be determined by approximating their point-spread function. (c) A super-resolved image is reconstructed from single-molecule coordinates
In a dSTORM experiment, single fluorophores are stochastically activated and read out over time, and an image stack is recorded. In each single image of the stack, the fluorescence emission pattern (or point-spread function) of single molecules is approximated with a Gaussian function and provides information on the location of a fluorophore. From a large number of single-molecule coordinates, an artificial image can be reconstructed (exemplary dSTORM images are shown in Fig. 2). As a result, dSTORM and similar methods provide a list of single-molecule coordinates, which is in contrast to conventional microscopy techniques which provide intensity information directly. In addition to generating super-resolution images and resolving cellular structures, this technique allows developing analytical localization-based algorithms to extract single-molecule information.
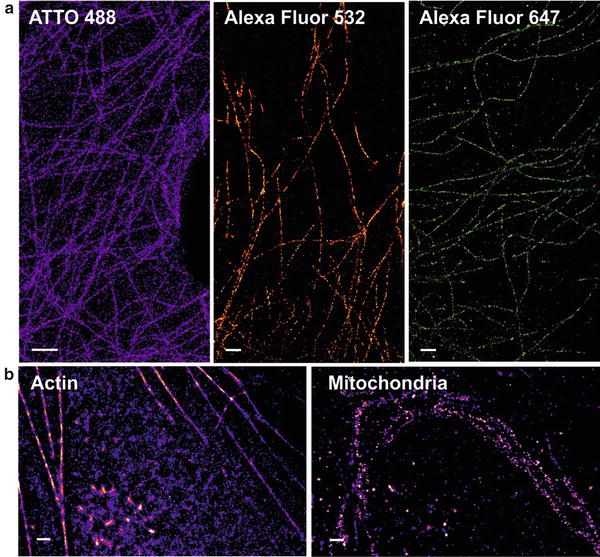
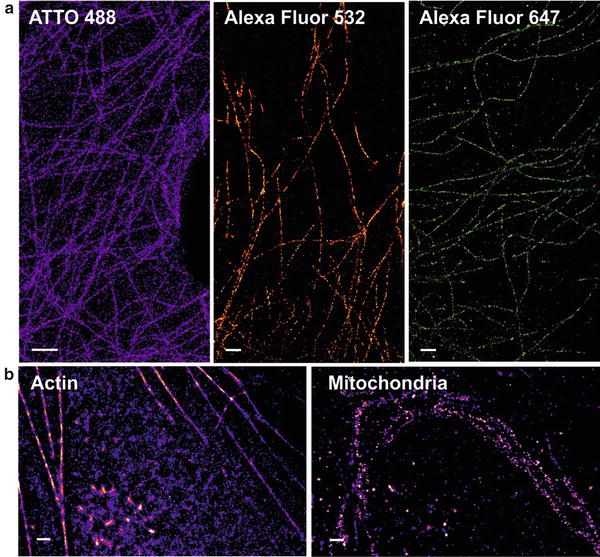
Fig. 2
dSTORM images of cellular structures. (a) Exemplary dSTORM images of microtubulin immunostained with different fluorophores. (b) Dual-color measurement of a cell stained for actin (EosFP) and mitochondria (Alexa Fluor 647). Scale bars represent 1 μm
2 Materials
dSTORM utilizes conventional organic fluorophores for super-resolution fluorescence microscopy, which are “programmed” to become photoswitches in the presence of reducing agents. The main classes of common organic fluorophores that can be photoswitched are carbocyanines [2], rhodamines, and oxazines [15].
3 Methods
Here we describe the basic approach for measuring dSTORM images in two dimensions in a static sample. It is possible to extend these methods for imaging dynamic samples, multiple colors, and three-dimensional structures (see Notes 1 – 3 ).
3.1 Labeling Strategy
Organic fluorophores are available as active esters and can be chemically conjugated to various functional groups found in biomolecules (e.g., –NH2 or –SH). This strategy enables labeling of small receptor-binding peptides, antibodies and proteins, oligonucleotides (e.g., molecular beacons), or small specific drug molecules (e.g., the actin-binding peptide phalloidin) as well as other substrates.
The most common labeling strategies for cellular structures that are compatible with dSTORM are:
1.
Immunocytochemistry: two complementary antibodies are applied as sandwich system, with a primary antibody targeting a specific antigen and a secondary antibody (or F(ab)2 fragment) carrying a fluorophore and targeting the first antibody.
2.
Chemical tags: The introduction of a chemical tag like the TMP tag [16], the SNAP tag [17], the CLIP tag [18], and the Halo tag [19] represents a simple, live cell compatible method for target-specific labeling with organic fluorophores. The protein of interest is genetically fused to a marker protein, which then covalently or non-covalently binds to a fluorescent tag.
3.
Click chemistry: The basic chemical reaction that underlies “click chemistry” is a Cu(I)-catalyzed cycloaddition of an azide and an alkyne to a triazole. This approach is very specific, as neither of the reactive groups is found in biological samples. Cells are treated with an azide or alkyne analogon (e.g., 5-ethynyl-2′-deoxyuridine (EdU) instead of thymidine for DNA labeling, or l-homopropargylglycine (HPG) or l-azidohomoalanine (AHA) for amino acid labeling), which is incorporated into biomolecules and subsequently labeled with a fluorophore carrying an alkyne or azide group [20, 21].
3.2 Photoswitching of Organic Fluorophores
Redox-induced photoswitching is best visualized and explained with a Jablonski diagram (Fig. 3). A fluorophore is first excited from the ground state (S0) into a higher electronic state (S1, S2, …, S N ). After internal conversion and vibrational relaxation to the lowest excited state (S1), spontaneous emission of a photon (fluorescence) brings the molecule back into the ground state S0. Alternatively, a non-radiative transition into S0 can occur, as well as intersystem crossing into the triplet state (T1).
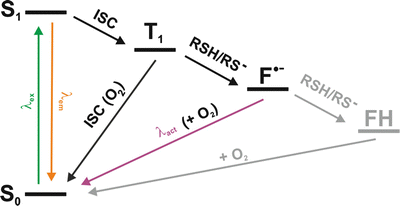
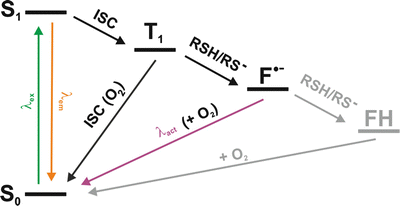
Fig. 3
Reversible photoswitching of organic fluorophores in the presence of reducing agents. Upon irradiation, the fluorophore is excited from its singlet ground state S0 into higher electronic states. From the first excited state S1, either fluorescence emission or intersystem crossing into the triplet state T1 occurs. The long-lived triplet state can further react with molecular oxygen to recover the singlet ground state or react with reducing agents (such as thiols) to form a radical anion (F•−). The singlet ground state can be recovered by oxidization with oxygen or excitation of the radical with near-UV light. For some fluorophores (e.g., the oxazine fluorophore ATTO 655) were found to become fully reduced to the leuco-form (FH), which can also recover into ground state by reaction with oxygen
In the presence of reducing agents which match the redox potential of the fluorophores, an electron transfer can occur. In the presence of thiol group containing reducing agents, many fluorophores are reduced out of the triplet state and form stable radical anions [22]. This radical state represents the nonfluorescent OFF-state of the fluorophore, which was demonstrated to exhibit a thermal stability of many minutes to hours in aqueous solution [22]. Upon reaction with molecular oxygen or irradiation with UV light, the molecule returns to the ground state, and fluorescence is recovered. The radical anion of some fluorophores (e.g., ATTO 655) exhibits a high electron affinity and can be further reduced to the leuco-form of a fluorophore [23].
The rate for the transition from the fluorescent ON-state into the nonfluorescent OFF-state (k off) is controlled by the concentration of the reducing thiol reagent and the irradiation intensity. The lifetime of the OFF-state and thus the rate of the transition into the ON-state (k on) depends on the thermal stability and the concentration of molecular oxygen. In addition, the OFF-state can be depopulated by irradiation with low-intensity near-UV light, which is in resonance with the absorption band of the radical anion of many fluorophores.
An important experimental parameter for high-quality dSTORM super-resolution imaging is an appropriate ratio of the ON- and OFF-switching rates, r = k off/k on. The higher the fluorophore density, the higher must be the ratio r, to ensure that each fluorophore is recorded as a single molecule at a given time. This implies rates k off ≫ k on which guarantee sufficiently low spot densities per imaging frame as the active fraction of fluorophores is low compared to the total number [24].
3.3 Microscope Setup
Localization-based super-resolution microscopy is a technique operated on microscopy setups with wide-field illumination (Fig. 4).
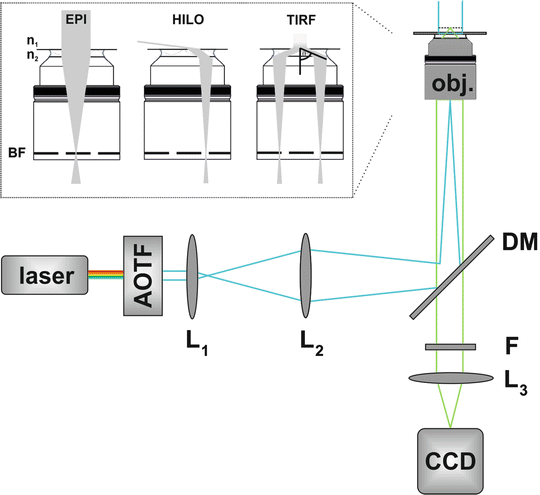
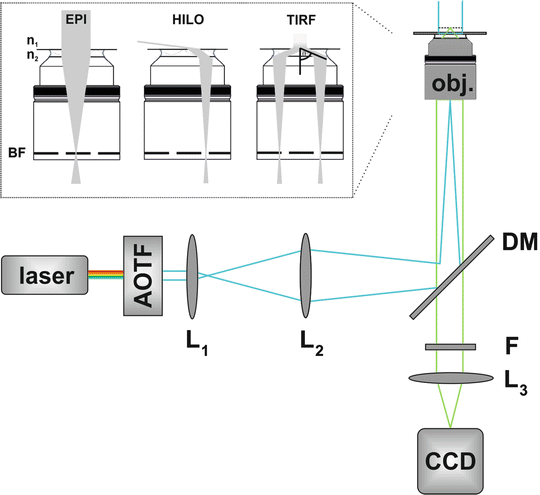
Fig. 4
Experimental setup for single-molecule super-resolution microscopy. An acousto-optical filter (AOTF) is used to select an excitation wavelength. The laser light is focused (e.g., by a telescope consisting of the lenses L1 and L2) onto the back focal (BF) plane of a high numerical aperture oil-immersion objective (OBJ). Excitation and emission light are separated by a dichroic mirror (DM). Fluorescence light is spectrally filtered by long-pass and/or bandpass filters (F) and projected (L3) on a camera. Additional lenses (not sketched) can be arranged in the detection path to adjust for a different pixel size
1.
Excitation pathway: a multiline laser (e.g., argon-krypton laser) or single laser sources (e.g., diodes or Ti:sapphire lasers) are filtered by an acousto-optic tunable filter to select for the appropriate excitation wavelength. The laser beam is then focused on the back focal aperture of the objective. A movable mirror enables to switch between wide-field illumination, total internal reflection (TIR) illumination, and highly inclined and laminated optical sheet (HILO) microscopy [25].
2.
Microscope configuration: an inverted microscope is equipped with an oil-immersion objective suitable for total internal reflection fluorescence microscopy (TIRFM). Excitation and fluorescence light are separated using a dichroic mirror.
3.
Detection pathway: the fluorescence light is directed onto a single-molecule sensitive electron-multiplying charge-coupled device (EMCCD) camera. Additional lenses may be introduced in the detection path to adjust for an optimal pixel size (70–160 nm) on the camera chip.
Examples for some commonly used fluorophores and appropriate filter sets are highlighted in Tables 1 and 2.
Table 1
Exemplary fluorophores suitable for dSTORM
Fluorophore | Absorption/emission (λ abs/λ em) | Switching buffer |
---|---|---|
Alexa Fluor 488 | 491/517 | 100 mM MEA, pH 8 |
ATTO 488 | 501/523 | 100 mM MEA, pH 9 |
Alexa Fluor 532 | 532/552 | 100 mM MEA, pH 7.4–8 |
Alexa Fluor 568 | 572/600 | 100 mM MEA, pH 8–8.5 |
Alexa Fluor 647/Cy5 | 649/670 | 100 mM MEA, pH 7.4 (+oxygen scavenger system) |
ATTO 655 | 663/684 | 10 mM MEA, pH 7.4–8 |
Table 2
Exemplary filter sets for single-color dSTORM measurements
Dyes | Cleanup | Dichroic mirror | Detection filter set |
---|---|---|---|
ATTO 488 | Z488/10, Chroma | HC-Quad 410/504/582/669, Semrock | LP 488 RU RazorEdge, Semrock |
Alexa Fluor 488 | HC 550/88 Brightline, Semrock | ||
ATTO 568 | Z568/10, Chroma | HC-Dual 560/659, Semrock | LP 568 RU RazorEdge, Semrock |
Alexa Fluor 568 | HQ 610/75, Chroma | ||
Cy5 | Z488/568/647 RPC, Chroma | HC-Dual 560/659, Semrock | LP 647 RU RazorEdge, Semrock |
Alexa Fluor 647 | ET 700/75, Chroma | ||
ATTO 655 |
3.4 dSTORM Imaging
Typically, a stack of 4,000–20,000 images with a frame rate between 10 Hz and 2 kHz is recorded. The EMCCD camera should be operated in the most sensitive mode, applying sufficient cooling for reduced thermal background noise, the highest dynamical range settings, and the fastest readout option by, e.g., a frame transfer mode and optimal gain settings. To achieve an optimal signal-to-noise ratio, the frame rate should be adjusted with respect to the average time a fluorophore resides in the fluorescent ON-state, which is controlled by the applied irradiation intensity. The appropriate recording time is dependent on the target (e.g., abundance and dimensionality), the labeling density, and the switching properties of the dye. The spot density, controlled by the transition rates between fluorescent “ON”- and nonfluorescent “OFF”-states, has to be sufficiently low for robust single-molecule detection. Furthermore, all fluorophores should be detected at least once, in order to allow for a proper visualization of a structure.
3.4.1 Drift Control
Due to the separation of single fluorophores in time, it is necessary to acquire enough images in order to reconstruct a representative super-resolution image, such that a dSTORM experiment typically lasts several minutes. As a consequence, localization-based super-resolution microscopy is very sensitive to drift occurring during the acquisition time. Small movements in the nanometer range can already introduce image artifacts and impact image quality and interpretation. Drift can be corrected for by adding photostable fluorescent beads as fiducial markers to the sample. Due to the stable and long-lasting fluorescence emission of the beads, the drift can be traced, and the individual frames of image stack can be realigned. Alternatively, image correlation can be used for drift correction, but requires a minimum of structural information.
3.5 Image Reconstruction
Single-molecule super-resolution methods such as dSTORM provide coordinates of individual point-spread functions recorded from single fluorophores. For each frame, the subset of active fluorophores needs to be identified and fitted precisely and rapidly using the post-processing software.
Commonly, the point spread function (PSF) of a single fluorophore is approximated by a Gaussian function:
with σ x and σ y as standard deviations, (x c, y c) the coordinates of the center, and the amplitude A and the background signal B.

Single-molecule localization software, like the freely available rapidSTORM [24, 26] and QuickPALM [27]), are based on two consecutive main tasks performed for each frame of the imaging stack:
1.
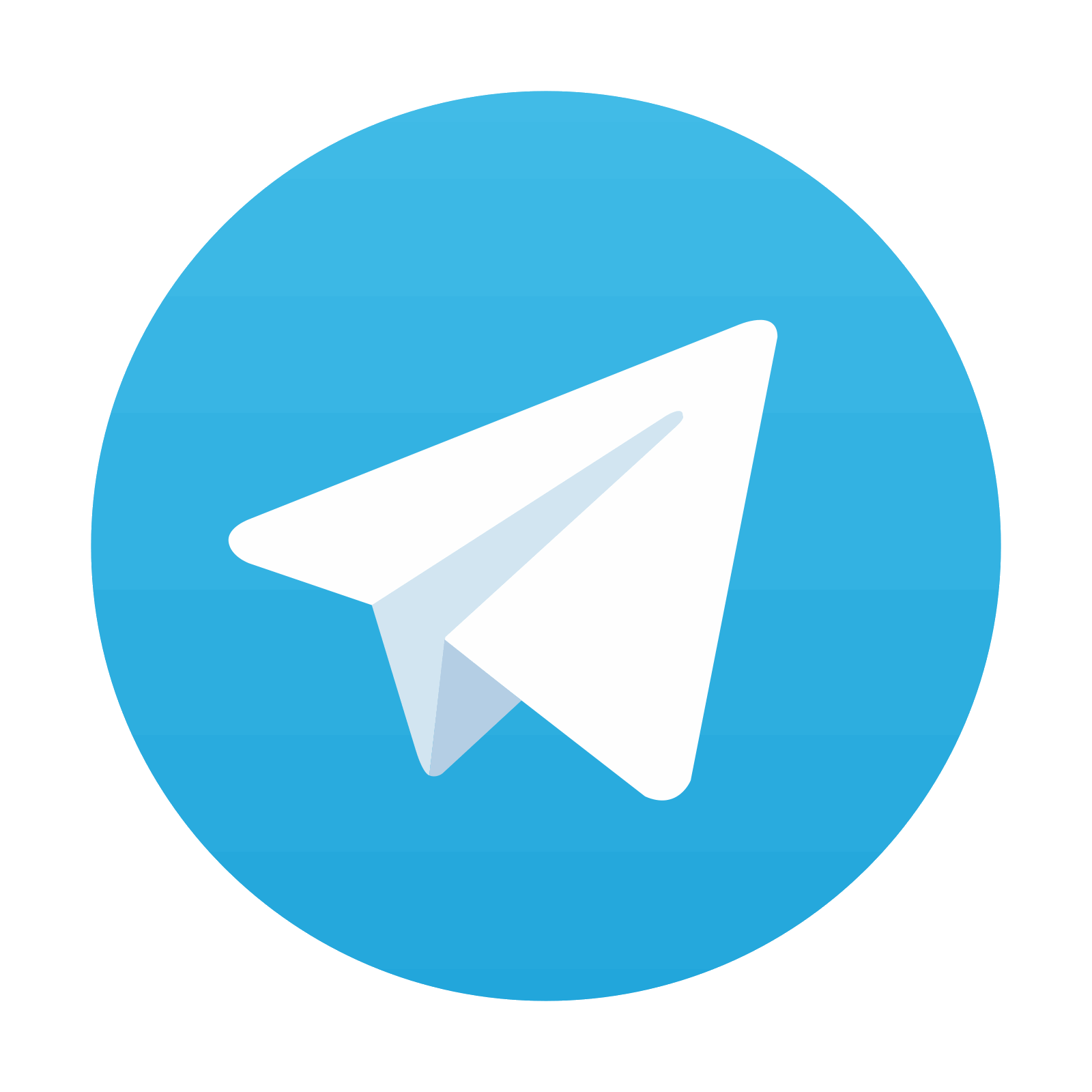
Spot identification: the PSF of single fluorophores is identified, e.g., by blurring and non-maximum suppression routines.
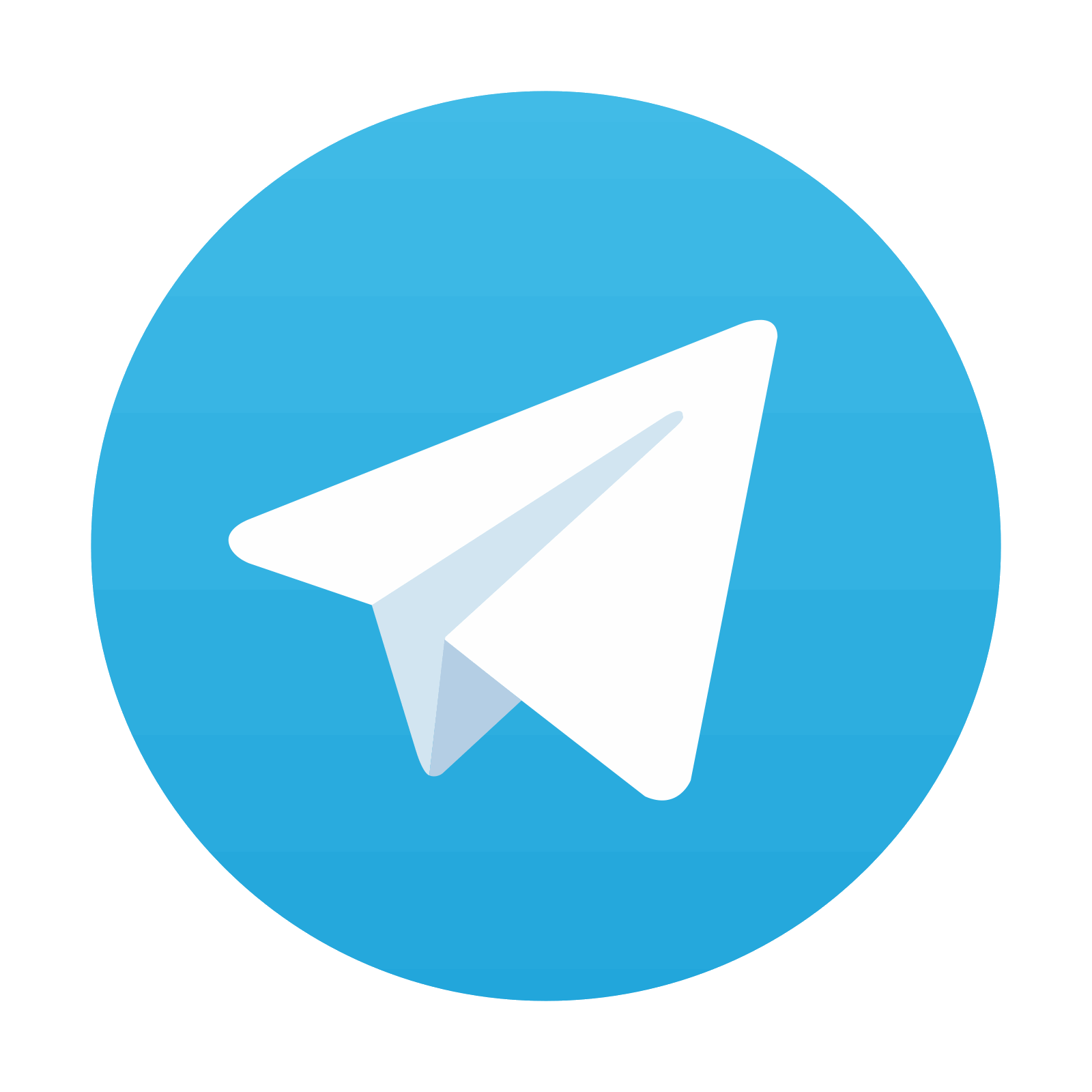
Stay updated, free articles. Join our Telegram channel
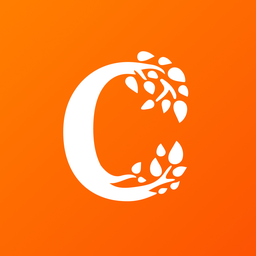
Full access? Get Clinical Tree
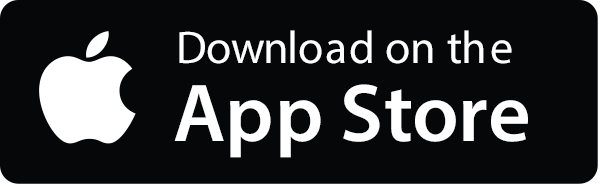
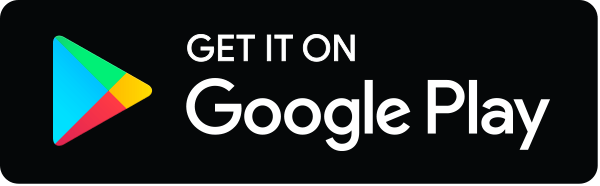
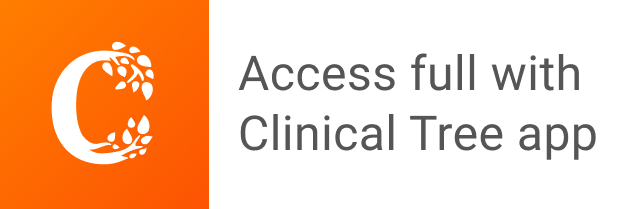