CHAPTER 24 Development of the nervous system
The entire nervous system and the special sense organs originate from three sources, each derived from specific cell populations of the early epiblast termed neural ectoderm. The first source to be clearly delineated is the neural plate, which gives rise to the central nervous system, the somatic motor nerves and the preganglionic autonomic nerves. The second source is from cells at the perimeter of the neural plate, neural crest cells, which remove themselves by epithelial/mesenchymal transition from the plate and migrate away just prior to its fusion into a neural tube. These cells give rise to the majority of the neurones and glia of the peripheral nervous system, i.e. the somatic sensory nerves, the somatic and autonomic ganglia, postganglionic autonomic nerves and suprarenal and chromaffin cells. They also give rise to significant mesenchymal populations in the head. The third source is from ectodermal placodes, which are focal thickening of the ectoderm covering the embryonic head. They contribute cells to the cranial sensory neurones, and form the olfactory epithelia, the epithelia of the inner ear and, by a non-neuronal contribution, the lens of the eye.
NEURULATION
Primary neurulation begins at stage 9 and is completed during stage 12 (Fig. 24.1). The process, although continuous spatially and temporally, has been envisaged as four stages. It begins with local elongation of the ectoderm cells in a midline zone of the embryonic disc and their reorganization into a pseudostratified epithelium, the neural plate. This is followed by reshaping and bending of the neural plate into a neural groove which subsequently closes to form into a neural tube bidirectionally from the midportion to its cranial and caudal ends. A continuous surface ectoderm forms dorsal to the tube.
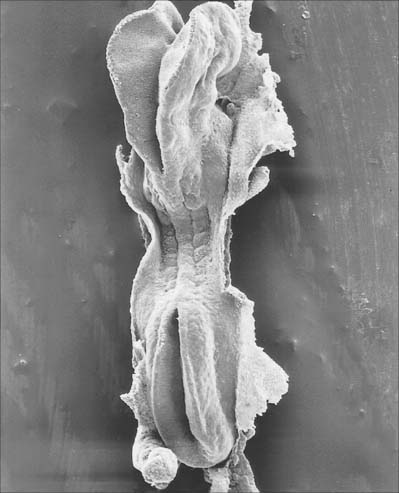
(Photograph by P Collins; printed by S Cox, Electron Microscopy Unit, Southampton General Hospital.)
Fusion of the neural tube starts in embryos with 4–6 somites, at the level of somites 1 and 2, forming the future rhombencephalon. The tube closes caudally and rostrally, forming sequentially cervical and thoracic cord regions, then mesencephalic and prosencephalic brain regions. Rostrally two sites of fusion can be seen. The initial fusion termed α, or the dorsal lip of the rostral neuropore, proceeds caudorostrally. A second site, termed β, or the terminal lip of the rostral neuropore, closes from the rostral end of the neural plate and proceeds rostrocaudally (O’Rahilly & Müller 2002). Closure of these lips of the rostral neuropore is completed when 19–20 pairs of somites are present. Caudal neuropore closure starts when approximately 29 somites are present and the site of closure corresponds to the level of the future somite 31 (the level of the future second sacral vertebra) (O’Rahilly & Müller 2004).
EARLY BRAIN REGIONS
Prior to the closure of the neural tube, the neural folds become considerably expanded in the head region; the first indication of a brain and its major divisions can be seen at stage 10 (Fig. 24.2). Regional expansions, prosencephalon (forebrain), mesencephalon (midbrain), and rhombencephalon (hindbrain), have been called the three primary cerebral vesicles, although the term ‘vesicle’ in this context has been considered inappropriate to describe localized accelerations of growth in the wall of the brain. As the neural tube closes, the neural wall appears to form a series of ridges and depressions perpendicular to its long axis. These transient repeating segments are termed primary neuromeres, and initially six can be identified: the prosencephalon, mesencephalon and four subdivisions of the rhombencephalon, rhombomeres A, B, C and D. The primary neuromeres themselves become subdivided during stages 10, 11 and 12 and a total of 16 secondary neuromeres have been identified. The prosencephalon gives rise to the telencephalon, diencephalon 1 (D1) and diencephalon 2 (D2), which has three subdivisions. The mesencephalon is subdivided into mesencephalon 1 (M1) and mesencephalon 2 (M2). The rhombencephalon is subdivided into the isthmus rhombencephali and rhombomeres 1–8; the original rhombomere A gives rise to secondary rhombomeres 1, 2 and 3; rhombomere B gives rise to secondary rhombomere 4; rhombomere C gives rise to secondary rhombomeres 5, 6 and 7; and rhombomere D gives rise to secondary rhombomere 8 (O’Rahilly & Müller 1999). As the rhombencephalon grows the rhombomeric boundaries become less distinct. With the early appearance of the cerebellum from the isthmus rhombencephali and rhombomere 1, the rhombocephalon is traditionally divided into the metencephalon, which extends to about rhombomere 3, and the myelencephalon, which extends to the spinomedullary junction. A summary of the derivative of the cerebral regions is given in Table 24.1 on page 376.
Table 24.1 Derivatives of the cerebral regions from caudal to rostral
Rhombencephalon (or hindbrain) | |
1. Myelencephalon | |
2. Metencephalon | |
3. Isthmus rhombencephali | |
Mesencephalon (or midbrain) | |
Prosencephalon (or forebrain) | |
1. Diencephalon | |
2. Telencephalon |
The elongation of the brain occurs at the same time as the appearance of three flexures, which also appear prior to the closure of the neural tube; two are concave ventrally and one concave dorsally. During stages 13 and 14 the brain bends at the mesencephalon (mesencephalic flexure) so that the prosencephalon bends in a ventral direction around the cephalic end of the notochord and foregut until its floor lies almost parallel with that of the rhombencephalon (Fig. 24.3). A bend also appears at the junction of the rhombencephalon and spinal cord (cervical flexure). This increases from the fifth to the end of the seventh week, by which time the rhombencephalon forms nearly a right angle to the spinal cord. However, after the seventh week, extension of the head takes place and the cervical flexure diminishes and eventually disappears. The third bend, the pontine flexure, is directed ventrally between the metencephalon and myelencephalon. It does not substantially affect the outline of the head. In this region, the roof plate thins until it is composed only of a single layer of cells and pia mater, the tela choroidea. The flexure of the neural tube at this point produces a rhombic shape in the roof which later forms the medullary velum.
EARLY CELLULAR ARRANGEMENT OF THE NEURAL TUBE
Histologically the early neural tube is composed of a pseudostratified neuroepithelium. It extends from the inner aspect of the tube to the outer limiting basal lamina and the surrounding pia mater. The epithelium contains stem cells which will give rise to populations of neuroblasts and glioblasts. A population of radial glia differentiates very early and provides a scaffold for later cells to follow. As development proceeds, three zones or layers develop (Figs 24.4–24.6). These are an internal ventricular zone (variously termed the germinal, primitive ependymal or matrix layer), in which mitosis occurs, and which contains the nucleated parts of the columnar cells and rounded cells undergoing mitosis; a middle, mantle zone (also termed the intermediate zone) which contains the migrant cells from the divisions occurring in the ventricular zone; and an outer, marginal zone, which initially consists of the external cytoplasmic processes of the radial glia. The latter is soon invaded by tracts of axonal processes which grow from neuroblasts developing in the mantle zone, together with varieties of non-neuronal cells (glial cells and later vascular endothelium and perivascular mesenchyme). For further development of these layers see pages 368–369.
At first the neural tube caudal to the brain is oval in transverse section and its lumen is narrow and slit-like (Fig. 24.4). The original floor plate and the dorsal site of fusion of the tube initially contain non-neural cells. With cellular proliferation, the lateral walls thicken and the lumen, now the central canal, widens in its dorsal part and is somewhat diamond-shaped on cross-section (Fig. 24.6). The widening of the canal is associated with the development of a longitudinal sulcus limitans on each side which divides the ventricular and mantle (intermediate) zones in each lateral wall into a ventrolateral lamina or basal plate and a dorsolateral lamina or alar plate respectively. This separation underlies a fundamental functional difference.
Throughout the neural tube there is a generic pattern in the position of the neurones which is specified by the juxtaposition of the notochord to the neural tube. Experimental lateral or dorsal grafting of a notochord results in the induction of a floor plate overlying the grafted notochord and the induction of ectopic motor neurones dorsally. Similarly, lateral or dorsal grafts of a floor plate also result in the induction of a new floor plate overlying the graft and the induction of ectopic dorsal motor neurones. Removal of the notochord results in the elimination of the floor plate and the motor neurones and the differentiation of dorsal cell types in the ventral region of the cord (Fig. 24.7).
FAILURE OF NEURULATION
Failure of neurulation produces the conditions of craniorachischisis totalis (where the entire neural tube is unfused in the dorsal midline), cranioschisis or anencephaly (where the neural tube is fused dorsally to form the spinal cord but is not fused dorsally in the brain), and spina bifida (where local regions of the spinal neural tube are unfused, or there is failure of formation of the vertebral neural arches). (Fig. 24.8; see also Ch. 44). Anencephalic fetuses display severe disturbances in the shape, position and ossification of the basichondrocranium and in the course of the intracranial notochord and so the condition is more complicated than ‘simply’ a failure of neural tube fusion.
NEURAL CREST
The neuronal populations of the early epiblast become arranged in the medial region of the embryonic disc as the neural plate. Laterally, neural folds or crests indicate the transitional region between neural and surface ectoderm. Along most of the neuraxis the cells at the tips of the neural folds undergo an epithelial/mesenchyme transformation. They acquire migratory properties and leave the epithelium just prior to its fusion with the contralateral fold in the dorsal midline. The migratory cells so formed are collectively termed the neural crest. Cells within the rostral prosencephalic neural fold and smaller populations of cells in bilateral sites lateral to the early brain do not form migratory neural crest cells but remain within the surface epithelium as ectodermal placodes.
Neural crest populations arise from the neural folds as primary neurulation proceeds and simultaneously progresses rostrally and caudally. Crest cells migrate from the neural folds of the brain prior to tube closure. Caudally, from approximately somite 29, secondary neurulation processes produce the most caudal neural crest. Two distinct populations of neural crest cells are formed: a neuronal population produced throughout the brain and spinal cord which gives rise to sensory and autonomic neurones and glia, and a non-neuronal mesenchymal population which arises only from the brain (Figs 24.9 and 24.10). Melanocytes develop from a subpopulation of neural crest cells derived from both the head and trunk. They form one of the three pigment cell types (the others being retinal pigment epithelium and the pigment cells of the pineal organ, which both originate from the diencephalon).
In the trunk the migration patterns of neural crest cells is channelled by the somites. As the crest cells move laterally and ventrally they can pass between the somites and within the rostral sclerotomal half of each somite, but they cannot penetrate the caudal moiety of the sclerotomal mesenchyme. Thus the segmental distribution of the spinal and sympathetic ganglia is imposed on the neural crest cells by a prepattern that exists within the somitic paraxial mesenchyme (Fig. 24.11). The origin of the cranial-caudal patterning of the ventral neural crest cells is not clear.
Rostral to the otic vesicle, neural crest cells arise from specific regions of the brain. Early in development, a number of transverse subdivisions perpendicular to the long axis of the brain can be seen within the rhombencephalon, dividing it into segments termed rhombomeres (Müller & O’Rahilly 1997). Eight main rhombomeres extend from the midbrain–hindbrain boundary rostrally to the spinal cord caudally (Fig. 24.2). Rhombomeres 8 and 7 give rise to neural crest cells which migrate into the fourth and sixth pharyngeal arches; rhombomere 6 crest cells invade pharyngeal arch three. Rhombomere 4 crest cells migrate into arch 2, whereas rhombomeres 5 and 3 give rise to a very small number of neural crest cells which migrate rostrally and caudally to enter the adjacent even-numbered neighbours. Rhombomeres 1 and 2 produce crest cells which invade the first pharyngeal arch. In each rhombomere, mesenchymal populations and the sensory and autonomic ganglia are formed from the crest cells (see Fig. 12.4).
ECTODERMAL PLACODES
Prior to neural tube closure, the elevating neural folds contain two distinctive neuronal populations. The larger population of neural crest cells migrates from the neural epithelium prior to neural tube fusion. A smaller population of neuroepithelial cells becomes incorporated into the surface ectoderm after neural tube closure. These areas of neuroepithelium within the surface ectoderm have been termed ectodermal placodes. Although the majority of the ectodermal placodes form nervous tissue, non-neurogenic placodes also occur (Begbie & Graham 2001). After an appropriate inductive stimulus, the placodes thicken and either generate migratory neuronal cells that will contribute to the cranial sensory ganglia, or the whole placodal region invaginates to form a vesicle beneath the remaining surface ectoderm. Neurogenic placodes undergo both processes. Paired non-neurogenic placodes invaginate to form the lens vesicles under the inductive influence of the optic vesicles (see Ch. 41).
The neural folds meet in the rostral midline adjacent to the buccopharyngeal membrane. This rostral neural fold does not generate neural crest but gives rise to the hypophysial placode, i.e. the future Rathke’s pouch, which remains within the surface ectoderm directly rostral to the buccopharyngeal membrane. The rostral neural fold also gives rise to the olfactory placodes, which remain as paired, laterally placed placodes, and to the epithelium of the nasal cavity (Fig. 24.10).
Further caudally, similar neurogenic placodes can be identified and divided into three categories, namely the epibranchial, otic and trigeminal placodes (Fig. 24.12). The epibranchial placodes appear in the surface ectoderm immediately dorsal to the area of pharyngeal (branchial) cleft formation. The first epibranchial placode is located at the level of the first pharyngeal groove and contributes cells to the distal (geniculate) ganglion of the facial nerve, the second and third epibranchial placodes contribute cells to the distal ganglia of the glossopharyngeal (petrosal) and vagus (nodose) nerves respectively. These placodes thicken and cells begin to detach from their epithelium soon after the pharyngeal pouches have contacted the overlying ectoderm. Concurrently the neural crest cells reach and move beyond these lateral extensions of the pharynx. Neurones migrate from the epibranchial placodes internally to the sites of ganglion formation, where they show signs of early differentiation into neurones, including the formation of neurites.
The otic placodes, located lateral to the myelencephalon, invaginate to form otic vesicles from which the membranous labyrinth of the ear develops. Neurones of the vestibulocochlear nerve ganglia arise from neurones that bud off the ventromedial aspect of the otic cup, after which they can be distinguished in the acoustic and vestibular ganglia (see Ch. 38).
PITUITARY GLAND (HYPOPHYSIS CEREBRI)
The most rostral portion of the neural plate, which will form the hypothalamus, is in contact rostrally with the future adenohypophysis in the rostral neural ridge, and caudally with the neurohypophysis, in the floor of the neural plate (Fig. 24.10). After neurulation the cells of the rostral neural ridge remain in the surface ectoderm and form the hypophysial placode which is in close apposition and adherent to the overlying prosencephalon.
Neural crest mesenchyme later moves between the prosencephalon and surface ectoderm except at the region of the placode. Before rupture of the buccopharyngeal membrane, proliferation of the periplacodal mesenchyme means that the placode forms the roof and walls of a saccular depression. This hypophysial recess (pouch of Rathke, Figs 24.13 and 24.14) is the rudiment of the adenohypophysis. It lies immediately ventral to the dorsal border of the buccopharyngeal membrane, extending in front of the rostral tip of the notochord, and retaining contact with the ventral surface of the prosencephalon. It is constricted by continued proliferation of the surrounding mesenchyme to form a closed vesicle, but remains for a time connected to the ectoderm of the stomodeum by a solid cord of cells, which can be traced down the posterior edge of the nasal septum. Masses of epithelial cells form mainly on each side and in the ventral wall of the vesicle, and the development of the adenohypophysis progresses by the ingrowth of a mesenchymal stroma. Differentiation of epithelial cells into stem cells and three differentiating types is said to be apparent during the early months of fetal development. It has been suggested that different types of cells arise in succession, and that they may be derived in differing proportions from different parts of the hypophysial recess. A craniopharyngeal canal, which sometimes runs from the rostral part of the hypophysial fossa of the sphenoid to the exterior of the skull, is often said to mark the original position of the hypophysial recess. Traces of the stomodeal end of the recess are usually present at the junction of the septum of the nose with the palate. Others have claimed that the craniopharyngeal canal itself is a secondary formation caused by the growth of blood vessels, and is quite unconnected with the stalk of the adenohypophysis.
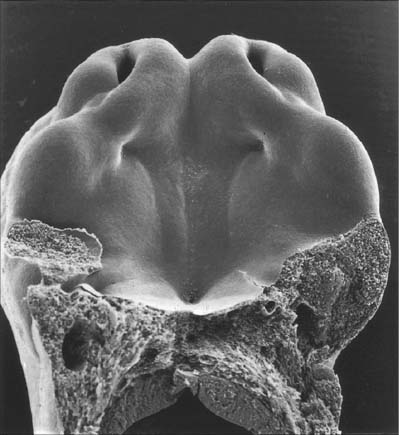
(Photograph by P Collins; printed by S Cox, Electron Microscopy Unit, Southampton General Hospital.)
Just caudal to, but in contact with, the adenohypophysial recess, a hollow diverticulum elongates towards the stomodeum from the floor of the neural plate just caudal to the hypothalamus (Fig. 24.14B); this region of neural outgrowth is the neurohypophysis. It forms an infundibular sac, the walls of which increase in thickness until the contained cavity is obliterated except at its upper end, where it persists as the infundibular recess of the third ventricle. The neurohypophysis becomes invested by the adenohypophysis, which extends dorsally on each side of it. The adenohypophysis gives off two processes from its ventral wall which grow along the infundibulum and fuse to surround it, coming into relation with the tuber cinereum and forming the tuberal portion of the hypophysis. The original cavity of Rathke’s pouch remains first as a cleft, and later as scattered vesicles, and can be identified readily in sagittal sections through the mature gland. The dorsal wall of Rathke’s pouch remains thin and fuses with the adjoining part of the neurohypophysis as the pars intermedia.
NEUROGLIA
The ventricular zone lining the early central canal of the spinal cord and the cavities of the brain gives rise to neurones and glial cells (Figs 24.4 and 24.5). One specialized form of glial cell is the radial glial cell, whose radial processes extend both outwards to form the outer limiting membrane deep to the pia mater, and inwards, to form the inner limiting membrane around the central cavity. The geometry of these cells may provide contact guidance paths for cell migrations, both neuronic and glioblastic. A secondary radial glial scaffold is formed in the late developing cerebellum and dentate gyrus and serves to translocate neurones, formed in secondary germinal centres, to their definitive adult locations. Radial glia eventually lose their connections with both inner and outer limiting membranes, except those persisting in the retina as Müller cells, in the cerebellum as Bergmann glia and in the hypothalamus as tanycytes. They can differentiate into neurones as well as astrocytes. They may partially clothe the somata of neighbouring developing neurones (between presumptive synaptic contacts), or similarly enwrap the intersynaptic surfaces of their neurites. Glial processes may expand around intraneural capillaries as perivascular end-feet. Other glioblasts retain an attachment (or form new expansions) to the pia mater, the innermost stratum of the meninges, as pial end-feet. Glioblasts also line the central canal and cavities of the brain as generalized or specialized ependymal cells, but lose their peripheral attachments. In some situations, as in the anterior median fissure of the spinal cord, ependymal cells retain their attachments to both the inner and outer limiting membranes. Thus, glia function as perineuronal satellites, and provide cellular channels interconnecting extracerebral and intraventricular cerebrospinal fluid, the cerebral vascular bed, the intercellular crevices of the neuropil and the cytoplasm of all neural cell varieties.
MECHANISMS OF NEURAL DEVELOPMENT
HISTOGENESIS OF THE NEURAL TUBE
The wall of the early neural tube consists of an internal ventricular zone (sometimes termed the germinal matrix) abutting the central lumen. It contains the nucleated parts of the pseudostratified columnar neuroepithelial cells and rounded cells undergoing mitosis. The early ventricular zone also contains a population of radial glial cells whose processes pass from the ventricular surface to the pial surface, thus forming the internal and external glia limitans (glial limiting membrane). As development proceeds the early pseudostratified epithelium proliferates and an outer layer, the marginal zone, devoid of nuclei but containing the external cytoplasmic processes of cells, is delineated. Subsequently a middle, mantle layer (intermediate zone) forms as the newly formed neurones migrate ventriculofugally from the ventricular zone (see Fig. 24.5).
Most CNS cells are produced in the proliferative zone adjacent to the future ventricular system, and in some regions this area is the only actively mitotic zone. According to the monophyletic theory of neurogenesis it is assumed to produce all cell types. The early neural epithelium, including the deeply placed ventricular mitotic zone, consists of a homogeneous population of pluripotent cells whose varying appearances reflect different phases in a proliferative cycle. The ventricular zone is considered to be populated by a single basic type of progenitor cell and to exhibit three phases. The cells show an ‘elevator movement’ as they pass through a complete mitotic cycle, progressively approaching and then receding from the internal limiting membrane (Fig. 24.15). DNA replication occurs while the cells are extended and their nuclei approach the pial surface; they then enter a premitotic resting period while the cells shorten and their nuclei pass back towards the ventricular surface. The cells now become rounded close to the internal limiting membrane and undergo mitosis. They then elongate and their nuclei move towards the outer edge during the postmitotic resting period, after which DNA synthesis commences once more and the cycle is repeated. The cells so formed may then either start another proliferative cycle or migrate outwards (i.e. radially) and differentiate into neurones as they approach and enter the adjacent stratum. This differentiation may be initiated as they pass outwards during the postmitotic resting period. The proliferative cycle continues with the production of clones of neurones and glioblasts. This sequence of events has been called inter-kinetic nuclear migration: it eventually declines. At the last division two postmitotic daughter cells are produced and they differentiate at the ventricular surface into ependyma.
The progeny of some of these divisions move away from the ventricular zone to form an intermediate zone of neurones. The early spinal cord and much of the brain stem shows only these three main layers, i.e. ventricular, intermediate and marginal zones. However, in the telencephalon the region of cellular proliferation extends deeper than the ventricular zone where the escalator movement of interkinetic migration is seen, and a subventricular zone appears between the ventricular and intermediate layers (Fig. 24.15). Here cells continue to multiply to provide further generations of neurones and glia which subsequently migrate into the intermediate and marginal zones. In some regions of the nervous system (e.g. the cerebellar cortex) some mitotic subventricular stem cells migrate across the entire neural wall to form a subpial population, and establish a new zone of cell division and differentiation. Many cells formed in this site remain subpial in position, but others migrate back towards the ventricle through the developing nervous tissue, and finish their migrations in various definitive sites where they differentiate into neurones or macroglial cells. In the cerebral hemispheres, a zone termed the cortical plate is formed outside the intermediate zone by radially migrating cells from the ventricular zone. The most recently formed cells migrate to the outermost layers of the cortical plate, so that earlier formed and migrating cells become subjacent to those migrating later. In the forebrain there is an additional transient stratum deep to the early cortical plate, the subplate zone.
LINEAGE AND GROWTH IN THE NERVOUS SYSTEM
Growth cones
During development, the growing axons of neuroblasts navigate with precision over considerable distances, often pursuing complex courses to reach their targets. Eventually they make functional contact with their appropriate end organs (neuromuscular endings, secreto-motor terminals, sensory corpuscles or synapses with other neurones). During the outgrowth of axonal processes the earliest nerve fibres are known to traverse appreciable distances over an apparently virgin landscape, often occupied by loose mesenchyme. A central problem for neurobiologists, therefore, has been understanding the mechanisms of axon guidance (Gordon-Weeks 2000). Axon guidance is thought to involve short-range, local guidance cues and long-range diffusible cues, any of which can be either attractive and permissive for growth, or repellent and hence inhibitory. Short-range cues require factors which are displayed on cell surfaces or in the extracellular matrix, e.g. axon extension requires a permissive, physical substrate, the molecules of which are actively recognized by the growth cone. They also require negative cues which inhibit the progress of the growth cone. Long-range cues come from gradients of specific factors diffusing from distant targets, which cause neurones to turn their axons towards the source of the attractive signal. The evidence for this has come from in vitro co-culture studies. The floor plate of the developing spinal cord exerts a chemotropic effect on commissural axons that later cross it, whereas there is chemorepulsion of developing motor axons from the floor plate. These forces are thought to act in vivo in concert in a dynamic process to ensure the correct passage of axons to their final destinations and to mediate their correct bundling together en route.
INDUCTION AND PATTERNING OF THE BRAIN AND SPINAL CORD
The regional pattern of the nervous system is induced before and during neural tube closure. Early concepts about regional patterning envisaged that regionalization within mesenchymal populations which transmit inductive signals to the ectoderm impose a similar mosaic of positional values on the overlying neural plate. For example, transplantation of caudal mesenchyme beneath the neural plate in Amphibia induced spinal cord, whereas rostral mesenchyme induced brain, as assessed by the morphology of the neuroepithelial vesicles. However, later work indicated a more complex scenario in which organizer grafts from early embryos induced mainly head structures, while later grafts induced mainly trunk structures. Subsequent molecular data have tended to support a model in which neural-inducing factors released by the organizer such as noggin, chordin and follistatin, neuralize the ectoderm and promote a mainly rostral neural identity. Later secreted signals then act to caudalize this rostral neural tissue, setting up an entire array of axial values along the neural tube. Candidates for these later, caudalizing, signals have been shown to be retinoic acid, fibroblast growth factors and the WNT secreted proteins, which are present in the paraxial mesenchyme and later in its derivatives, the somites. This combination of signals does not seem to be sufficient to produce the most rostral, forebrain structures. Other secreted proteins resident in the rostralmost part of the earliest ingressing axial populations of endoderm and mesenchyme are also capable of inducing markers of forebrain identity from ectodermal cells (Withington, Beddington & Cooke 2001).
As the neural tube grows and is modified in shape, a number of mechanisms refine the crude rostrocaudal pattern which has been imposed during neurulation. Molecules which diffuse from tissues adjacent to the neural tube such as the somites have patterning influences. The neural tube possesses a number of intrinsic signalling centres, such as the midbrain–hindbrain boundary, which produce diffusible molecules capable of influencing tissue development at a distance. In this way extrinsic and intrinsic factors serve to subdivide the neural tube into a number of fairly large domains, on which local influences can then act. Domains are distinguished by their expression of particular transcription factors, which in many cases have been causally related to the development of particular regions. Examples of such genes are the Hox family which are expressed in the spinal cord and hindbrain, and the Dlx, Emx and Otx families of genes which are expressed in various regions of the forebrain. All of these are developmental control genes which lie high up in the hierarchy, and are capable of initiating cascades of expression of other genes to create a more fine-grained pattern of cellular differentiation. In contrast to the aforementioned secreted molecules, these genes encode proteins which are retained in the cell nucleus, and so can act on DNA to induce or repress further gene expression.
Segmentation in the neural tube
The early neural tube is visibly divided into segments, termed neuromeres, by shallow transverse folds which extend perpendicular to its long axis. Primary neuromeres can be identified at stage 9, and 16 secondary neuromeres are present at stage 14. They are especially noted in the rhombencephalon, where they are termed rhombomeres; they have now been shown to constitute crucial units of pattern formation. Domains of expression of developmental control genes abut rhombomere boundaries; single cell labelling experiments have revealed that cells within rhombomeres form segregated non-mixing populations (Fig. 24.16). The neural crest also shows intrinsic segmentation in the rhombencephalon, and is segregated into streams at its point of origin in the dorsal neural tube. This may represent a mechanism whereby morphogenetic specification of the premigratory neural crest cells is conveyed to the pharyngeal arches (see Fig. 12.4). Although these segmental units lose their morphological prominence with subsequent development, they represent the fundamental ground plan of this part of the neuraxis, creating a series of semi-autonomous units within which local variations in patterning can then develop. The consequences of early segmentation for events later in development, such as the formation of definitive neuronal nuclei within the brain stem, and of peripheral axonal projections remain to be explored.
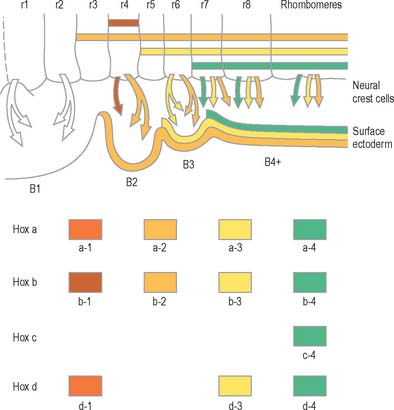
(Modified by permission from the Annual Review of Cell and Developmental Biology, Volume 8, 1992 by Annual Reviews www.annualreviews.org.)
Genes such as the Hox and Pax gene families, which encode transcription factor proteins, show intriguing expression patterns within the nervous system. Genes of the Hox-b cluster, for example, are expressed throughout the caudal neural tube, and up to discrete limits in the hindbrain that coincide with rhombomere boundaries. The ordering of these genes within a cluster on the chromosome (5′-3′) is the same as the caudal to rostral limits of expression of consecutive genes. This characteristic pattern is surprisingly similar in fish, frogs, birds and mammals. Hox genes play a role in patterning not only of the neural tube but also of much of the head region, consistent with their expression in neural crest cells, and within the pharyngeal arches. Disruption of Hox a-3 gene in mice mimics DiGeorge’s syndrome, a congenital human disorder characterized by the absence (or near absence) of the thymus, parathyroid and thyroid glands, by the hypotrophy of the walls of the arteries derived from the aortic arches, and by subsequent conotruncal cardiac malformations. Some Pax genes are expressed in different dorsoventral domains within the neural tube. Pax-3 is expressed in the alar lamina, including the neural crest, while Pax-6 is expressed in the intermediate plate. The Pax-3 gene has the same chromosomal localization as the mouse mutation Splotch and the affected locus in the human Waardenburg’s syndrome, both of which are characterized by neural crest disturbances with pigmentation disorders and occasional neural tube defects. Both Hox and Pax genes have restricted expression patterns with respect to the rostrocaudal and the dorsoventral axes of the neural tube, consistent with roles in positional specification. (For reviews of the expression patterns of these genes see Krumlauf et al 1993.)
While craniocaudal positional values are probably conferred on the neuroepithelium at the neural plate or early neural tube stage, dorsoventral positional values may become fixed later. The development of the dorsoventral axis is heavily influenced by the presence of the underlying notochord. The notochord induces the ventral midline of the neural tube, the floor plate. This specialized region consists of a strip of non-neural cells with distinctive adhesive and functional properties. Notochord and floor plate together participate in inducing the differentiation of the motor columns. Motor neurone differentiation occurs early, giving some grounds for the idea of a ventral to dorsal wave of differentiation. The notochord/floor plate complex may also be responsible for allotting the values of more dorsal cell types within the tube (Fig. 24.7). For example, the dorsal domain of expression of Pax-3 extends more ventrally in embryos experimentally deprived of notochord and floor plate, while grafting an extra notochord adjacent to the dorsal neural tube leads to a repression of Pax-3 expression.
PERIPHERAL NERVOUS SYSTEM
SOMATIC NERVES
Spinal nerves
Each spinal nerve is connected to the spinal cord by a ventral root and a dorsal root (Fig. 24.17). The fibres of the ventral roots grow out from cell bodies in the anterior and lateral parts of the intermediate zone. These pass through the overlying marginal zone and external limiting membrane. Some enter the myotomes of the somites, and some penetrate the somites, reaching the adjacent somatopleure, and in both sites they ultimately form the α-, β- and γ-efferents. At appropriate levels these are accompanied by the outgrowing axons of preganglionic sympathetic neuroblasts (segments T1–L2), or preganglionic parasympathetic neuroblasts (S2–S4).
The fibres of the dorsal roots extend from cell somata in dorsal root ganglia (DRG) into the spinal cord and also extend into the periphery. Neural crest cells are produced continuously along the length of the spinal cord, but gangliogenic cells migrate only into the rostral part of each somitic sclerotome where they condense and proliferate to form a bilateral series of oval-shaped primordial spinal ganglia (dorsal root ganglia) (Fig. 24.11). Negative factors in the caudal sclerotome deter neural crest from entering. The rostral sclerotome has a mitogenic effect on the crest cells that settle within it. From the ventral region of each ganglion a small part separates to form sympathochromaffin cells, while the remainder becomes a definitive spinal ganglion (dorsal root ganglion). The spinal ganglia are arranged symmetrically at the sides of the neural tube and, except in the caudal region, are equal in number to the somites. The cells of the ganglia, like the cells of the intermediate zone of the early neural tube, are glial and neuronal precursors. The glial precursors develop into satellite cells (which become closely applied to the ganglionic nerve cell somata), Schwann cells, and possibly other cells. The neuroblasts, at first round or oval, soon become fusiform, and their extremities gradually elongate into central and peripheral processes. The central processes grow into the neural tube as the fibres of dorsal nerve roots, while the peripheral processes grow ventrolaterally to mingle with the fibres of the ventral root, thus forming a mixed spinal nerve. As development proceeds the original bipolar form of the cells in the spinal ganglia changes and the two processes become approximated until they ultimately arise from a single stem to form a unipolar cell. The bipolar form is retained in the ganglion of the vestibulocochlear nerve.
Cranial nerves
Cranial nerves may contain motor, sensory or both types of fibres. With the exception of the olfactory and optic nerves, the cranial nerves develop in a manner similar in some respects to components of the spinal nerves. The somata of motor neuroblasts originate within the neuroepithelium, while those of sensory neuroblasts are derived from the neural crest with the addition in the head of contributions from ectodermal placodes (Fig. 24.18; see Fig. 12.4).
In the head the motor outflow is traditionally segregated into two pathways (Figs 24.3B and 24.18). General somatic efferent neurones exit ventrally in a similar manner to those of the spinal cord. Thus the oculomotor, trochlear, abducens and hypoglossal nerves parallel the organization of the somatic motor neurones in the spinal cord. The second motor component, special branchial efferent, consists of the motor parts of the trigeminal, facial, glossopharyngeal and vagus nerves which supply the pharyngeal (branchial) arches, and the accessory nerve. These nerves all have nerve exit points more dorsally placed than the somatic motor system.
These motor neurone types have been designated according to the types of muscles or structures they innervate. General somatic efferent nerves supply striated muscle derived from the cranial (occipital) somites and prechordal mesenchyme. Myogenic cells from the ventrolateral edge of the epithelial plate of occipital somites give rise to the intrinsic muscles of the tongue, while the prechordal mesenchyme gives rise to the extrinsic ocular muscles. Special branchial efferent nerves supply the striated muscles developing within the pharyngeal (branchial) arches (see Fig. 12.4) which are derived from parachordal mesenchyme between the occipital somites and the prechordal mesenchyme. All the voluntary muscles of the head originate from axial (prechordal) or paraxial mesenchyme which renders the distinction between somatic efferent supply and branchial efferent supply somewhat artificial. However, the obviously special nature of the arch musculature, its patterning by the neural crest cells, its particularly rich innervation for both voluntary and reflex activity, and the different origins from the basal plate of the branchial efferent nerves compared to the somatic efferent nerves, make the retention of a distinction between the two of some value.
The cranial sensory ganglia are derived in part from the neural crest, and in part from cells of the ectodermal placodes (Figs 24.12 and 24.18). Generally, neurones distal to the brain are derived from placodes while proximal ones are derived from the neural crest (Fig. 24.18). Supporting cells of all sensory ganglia arise from the neural crest. The most rostral sensory ganglion, the trigeminal, contains both neural crest and placode-derived neurones that mediate general somatic afferent functions. In the case of more caudal cranial nerves (the facial, glossopharyngeal and vagus), the same applies, but the two cell populations form separate ganglia in the case of each nerve. The proximal series of ganglia is neural crest derived (forming the proximal ganglion of the facial nerve, the superior ganglion of the glossopharyngeal nerve and the jugular ganglion of the vagus) while the distal series is derived from placodal cells (forming the geniculate ganglion of the facial nerve, the petrosal ganglion of the glossopharyngeal nerve and the nodose ganglion of the vagus). These ganglia contain neurones that mediate special, general visceral and somatic afferent functions. The vestibular ganglion contains both crest and placodal cells and the acoustic ganglion contains only placodal neurones: the axons from these cells are special somatic afferents and they all travel in the vestibulocochlear nerve.
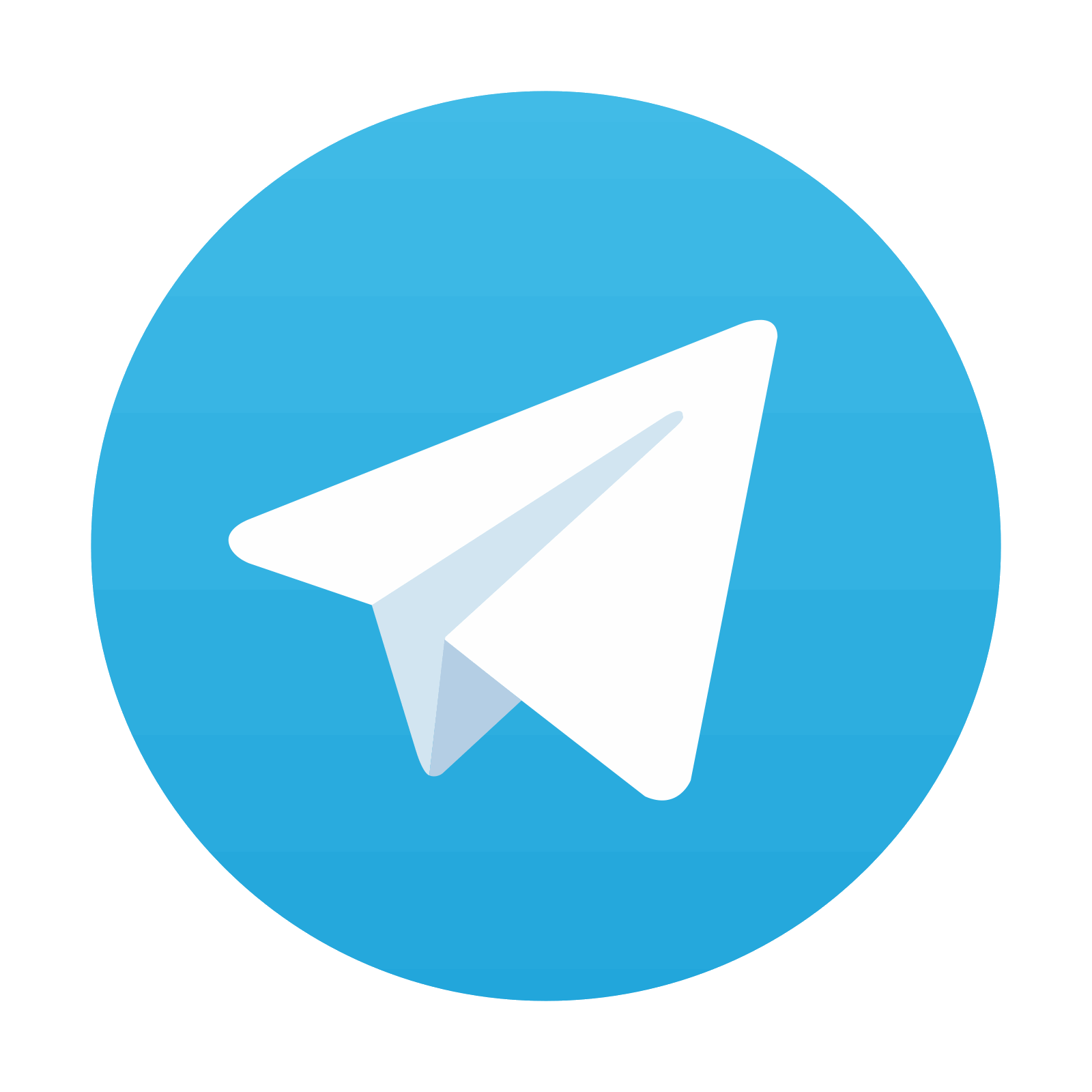
Stay updated, free articles. Join our Telegram channel
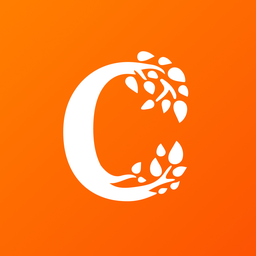
Full access? Get Clinical Tree
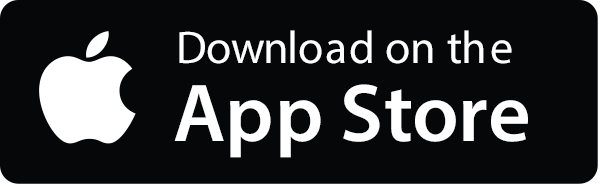
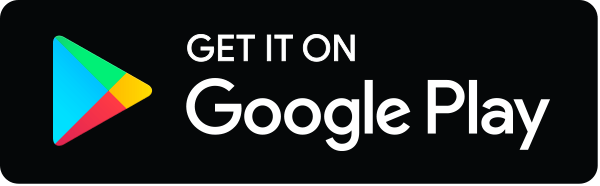