and Jürgen Roth2
(1)
Medical University of Vienna, Vienna, Austria
(2)
University of Zurich, Zurich, Switzerland
Secretory Pathway of Pancreatic Acinar Cells
In their classical Nobel Prize–winning studies on protein biosynthesis and secretion, Palade and coworkers used the exocrine pancreas as a model tissue. The low-power electron micrograph from rat exocrine pancreas shows acinar cells and immunogold labeling for one of their major secretory products, the digestive enzyme amylase. As such, it provides a general idea of the main structural elements of the secretory pathway in a highly specialized secretory cell. The immunoelectron microscopic demonstration of intracellular antigens in ultrathin sections has been critically improved by the introduction of gold particles as a marker. The scheme shows the two-step protein A-gold technique to detect antigenic sites in ultrathin sections.


Several acinar cells assemble to form a structural and functional unit, a so-called acinus, which is the main component of the gland parenchyma (cf. Figs. 1 and 111).
The rough endoplasmic reticulum (RER), which is abundant in the basal and juxtanuclear portions of the pancreatic acinar cells and other secretory cell types, represents the first structural element of the secretory pathway, sometimes also referred to as the endomembrane system. It shows abundant immunogold labeling for amylase and the gold particles appear as black spots. The next major element is represented by the Golgi apparatus, which can be seen in its typical supranuclear location and which is intensely immunogold labeled for amylase. The pre-Golgi intermediates cannot be recognized at this low magnification. The supranuclear cytoplasm contains numerous secretory granules in which amylase and many other secretory products are stored. The secretory granules of the acinar cells are called zymogen granules (ZG) because of their content of proenzymes. On appropriate stimulation, the zymogen granules fuse with the apical plasma membrane and discharge their content into the acinus lumen (AL). This stimulus-mediated exocytosis is called regulated secretion (cf. Fig. 54). The acinus lumen (AL) represents the first part of the extracellular excretory duct system.
A detailed account of the structural and functional aspects of the secretory system is given in the following plates.
M: mitochondria
References
Palade G (1975) Intracellular aspects of the process of protein biosynthesis. Science 189:347
Roth J (1981) The colloidal gold marker system for light and electron microscopic cytochemistry. In: Bullock GR, Petrusz P (eds) Techniques in immunocyto-chemistry, vol 2. Academic, London, p 217
Roth J (1989) Postembedding labeling on Lowicryl K4M tissue sections: detection and modification of cellular components. In: Tartakoff AM (ed), Vesicular Transport, Part B, Meth Cell Biol. Academic Press, San Diego, p 513
Roth J, Bendayan M, Orci L (1978) Ultrastructural localization of intracellular antigens by the use of protein A-gold complex. J Histochem Cytochem 26:1074
Endomembrane System of Dinoflagellates
Although the various organelles of mammalian cells are in the focus of this book, it should be noted that unicellular organisms have provided invaluable information about the biogenesis, molecular structure, and function of various cellular organelles because their principles have been found to be phylogenetically highly conserved. As a case in point, studies in the yeast Saccharomyces cerevisiae were of fundamental importance for the molecular dissection of the secretory pathway and of autophagy. In wild-type Saccharomyces cerevisiae, however, elements of the rough endoplasmic reticulum are scarce and stacks of flat cisternae building a Golgi apparatus resembling that of mammalian cells are rarely found. However, in sec7 and sec14 secretory mutants, Golgi apparatus composed of cisternal stacks could be readily observed at the nonpermissive temperature. Other unicellular organisms such as the parasitic protists contain a comparatively well-developed endomembrane system.
The marine dinoflagellates represent other unicellular organisms with a highly developed endomembrane system, as shown in panel A. For high contrast and distinct appearance of cellular membranes, the cells were fixed in reduced osmium tetroxide after initial double aldehyde fixation. It is notable that, by applying this fixation protocol, ribosomes can be barely recognized. In panel A, an equatorially sectioned dinoflagellate is shown, which contains a prominent nucleus and numerous plastids in its periphery. The many mitochondria are of tubulus type (cf. Fig. 77), and several cisternae of the endoplasmic reticulum can be seen. In panel B, a site of continuity between the nuclear envelope (NE) and endoplasmic reticulum is indicated by an arrowhead. In close relationship with a curved cisterna of the endoplasmic reticulum (ER), a membrane cluster composed of many tubules and vesicles is visible that most probably represents a peripheral pre-Golgi intermediate (cf. Figs. 29, 30, 31). In panel C, a Golgi apparatus cisternal stack is shown with numerous associated vesicles at either of its poles and the lateral extremities. Vesicles and short tubules are also located between a presumptive transitional element of the endoplasmic reticulum (ER) and a fenestrated cis Golgi cisterna. Such solid stacks of flattened cisternae appear to be linked by fenestrated elements and tubules, as also seen in mammalian cells. The arrowhead in panel C marks a (clathrin)-coated lateral extension of a trans Golgi cisterna.
References
Grell KG, Wohlfarth-Bottermann KE (1957) Light- and electron microscopy of the Dinoflagellate Amphidinium elegans n. sp. Z Zellforsch Mikrosk Anat 47:7
Kaiser CA, Schekman R (1990) Distinct sets of SEC genes govern transport vesicle formation and fusion early in the secretory pathway. Cell 61:723
Novick P, Field C, Schekman R (1980) Identification of 23 complementation groups required for post-translational events in the yeast secretory pathway. Cell 21:205
Rambourg A, Daraspe J, Képès F, Verbavatz J-M (2008) Morphodynamics of the yeast Golgi apparatus. In: Mironov AA, Pavelka M (eds) The Golgi apparatus. State of the art 110 years after Camillo Golgi’s discovery. Springer, Wien/NewYork, p 631
Sokolova YY, Mironov AA (2008) Structure and function of the Golgi organelle in parasitic protists. In: Mironov AA, Pavelka M (eds) The Golgi apparatus. State of the art 110 years after Camillo Golgi’s discovery. Springer, Wien/NewYork, p 647
Taylor FJ (1980) On dinoflagellate evolution. Biosystems 13:65
Warren G (2013) Transport through the Golgi in Trypanosoma brucei. Histochem Cell Biol 140:235



Fig. 17
Magnification: ×15,500

Fig. 18
Magnification: ×7,250 (A); ×41,500 (B, C)
Ribosomes, Rough Endoplasmic Reticulum
Ribosomes are RNA-protein particles measuring 30 nm at their largest dimensions, which function as machineries for protein synthesis. Within a ribosome, a messenger-RNA (mRNA) and transfer-RNAs (tRNA) are brought together, thus allowing base pairing between mRNA codons and tRNA anticodons, which drives the synthesis of a polypeptide with an amino acid sequence according to the model specified by the mRNAs codons.
Ribosomes are composed of two subunits, which are formed in the nucleolus. Co- and posttranscriptionally, the preribosomal RNA (pre-rRNA) encoded by the ribosomal DNA (rDNA) associates with small nucleolar RNAs (snoRNAs) and non-ribosomal proteins to be modified and processed. A series of cleavages takes place, leading to the 18, 28 and 5.8S rRNAs, which together with ribosomal proteins and the 5S rRNA synthesized outside the nucleolus, assemble to form the small and large precursors of the 40 and 60S ribosomal subunits. They are separately exported out of the nucleus through the nuclear pore complex (NPC), the large precursor utilizing the adaptor protein Nmd3 for binding to the Crml nuclear export factor. Ribosomes exist as distinct particles only during ongoing protein synthesis. The 40 and 60S subunits assemble during the protein synthesis initiation phase and again dissociate and enter a common cytoplasmic pool after completion of the protein.
Polysomes are ribosome clusters attached to one mRNA and engaged in active translation; several copies of the same protein are produced simultaneously. Depending on the class of proteins synthesized, polysomes either reside “freely” in the cytoplasm (“free polysomes”) or bind to membranes of the rough endoplasmic reticulum (RER, “bound polysomes”). The latter process is receptor-mediated and guided by a signal peptide, which is part of all proteins to be synthesized at the RER.
Panels A and B show details of cells extensively involved in synthesis of secretory proteins. The basal cytoplasm of a pancreatic acinar cell shown in panel A contains densely packed, flat RER-cisternae forming a cellular compartment called ergastoplasm (“busy plasma”). The membranes are studded with ribosomes (arrowheads) and fine filamentous materials are contained in the cisternal lumen, mainly corresponding to newly synthesized secretory proteins. Polysomes appear as “rosettes,” strings of beads in single or double rows (arrowheads in panel B) or serpent-like structures (arrows in panel B). Studies of native cytosolic polysomes in intact cells by cryo-electron tomography combined with computational methods showed an orientation of the ribosomes in such a way that the small subunits are brought into close proximity and the large subunits are kept apart.
RER-bound ribosomes also produce luminal and membrane proteins of the entire secretory system, lysosomal enzymes, and plasma membrane proteins. In contrast, ribosomes do not bind to the RER during synthesis of other proteins, such as proteins residing at the cytoplasmic side of membranes, cytoskeletal proteins, and proteins destined to be transported into the nucleus or inserted into mitochondria or peroxisomes. The RER is organized into subregions (cf. also Fig. 20) and findings suggest that particular mRNAs encoding for secretory or membrane proteins are confined to distinct areas of the RER. There is evidence for transport of mRNAs along either microtubules or actin filaments, and several RER-associated proteins involved in anchoring mRNAs have been identified.
References
Baumann O, Walz B (2001) Endoplasmic reticulum of animal cells and its organization into structural and functional domains. Int Rev Cytol 205:149
Brandt F, Carlson L-A, Hartl U, Baumeister W, Grünewald K (2010) The three-dimensional organization of polyribosomes in intact human cells. Mol Cell 39:560
Nikonov AV, Snapp E, Lippincott-Schwartz J, Kreibich G (2002) Active translocon complexes labeled with GFP-Dadl diffuse slowly as large polysome arrays in the endoplasmic reticulum. J Cell Biol 158:497
Olson MOJ, Hingorani K, Szeneni A (2002) Conventional and nonconventional roles of the nucleolus. Int Rev Cytol 219:199
Park SH, Blackstone C (2010) Further assembly required: construction and dynamics of the endoplasmic reticulum network. EMBO Rep 11:515
Shibata Y, Shemesh T, Prinz WA, Palazzo AF, Kozlov MM, Rapoport TA (2010) Mechanisms determining the morphology of the peripheral ER. Cell 143:774
Wong AK, Chao JT, Loewen CJR (2014) Barriers to uniformity within the endoplasmic reticulum. Curr Opin Cell Biol 29:31


Fig. 19
Magnification: ×52,000 (A), ×46,000 (B)
Nuclear Envelope and Rough Endoplasmic Reticulum
The nuclear envelope (NE) consists of an inner and an outer membrane enclosing the perinuclear cisterna or perinuclear space. The outer nuclear membrane is studded with ribosomes and continuous with the membrane of the rough endoplasmic reticulum (RER). Therefore, direct continuity exists between the lumen of the nuclear envelope and the rough endoplasmic reticulum, as marked by lines in panel A. Evidence is ample that the outer nuclear membrane is engaged in protein synthesis and that de novo synthesized proteins are translocated in the perinuclear space. Posttranslational modifications on proteins may occur here because of the presence of oligosaccharide-trimming glycosidases (cf. Fig. 23).
Although the rough endoplasmic reticulum and the nuclear envelope form a continuous network, functional domains seem to exist in this interconnected open system. As an example, the heterogeneous distribution of apomucin in mucus cells of submandibular glands is presented. Mucin is the main secretory product in this cell type and can be readily detected by immunogold electron microscopy. In panel A, immunogold labeling for apomucin (non-glycosylated mucin) in the cisternal lumen of the rough endoplasmic reticulum is evident. However, the perinuclear cistern even at sites of continuity with the cisternal lumen of the rough endoplasmic reticulum is unlabeled. In panel B, intensely apomucin-positive parts of cisternae of rough endoplasmic reticulum can be seen alternating with parts that are completely unlabeled (marked by lines). This represents a remarkable example of segregation of a major secretory product in the lumen of the continuous network of rough endoplasmic reticulum cisternae. As for other reported examples, it is currently unknown how such domains become established and are maintained. It has been proposed that the endoplasmic reticulum is a site of mRNA localization that will associate for its lifetime with membrane-bound polyribosomes. As a result, the synthesis of the respective proteins will be compartmentalized.
References
Chen S, Novick P, Ferro-Novik S (2013) ER structure and function. Curr Opin Cell Biol 25:428
Deschuyteneer M, Eckhardt AE, Roth J, Hill RL (1988) The subcellular localization of apomucin and nonreducing terminal N-acetylgalactosamine in porcine submaxillar glands. J Biol Chem 263:2452
English AR, Voeltz GK (2013) Endoplasmic reticulum structure and interconnections with other organelles. Cold Spring Harb Perspect Biol. doi:10.1101/cshperspect.a013227
Hetzer MW (2010) The nuclear envelope. Cold Spring Harb Perspect Biol 2:a000539
Nicchitta CV (2002) A platform for compartmentalized protein synthesis: protein translation and translocation in the ER. Curr Opin Cell Biol 14:412
Prunuske AJ, Ullman KS (2006) The nuclear envelope: form and reformation. Curr Opin Cell Biol 18:108
Shibata Y, Hu J, Kozlov MM, Rapoport TA (2009) Mechanisms shaping the membranes of cellular organelles. Annu Rev Cell Dev Biol 25:329
Sitia R, Meldolesi J (1992) Endoplasmic reticulum – a dynamic patchwork of specialized subregions. Mol Biol Cell 3:1067
Spiliotis ET, Pentcheva T, Edidin M (2002) Probing for membrane domains in the endoplasmic reticulum: retention and degradation of unassembled MHC class I molecules. Mol Biol Cell 13:1566


Fig. 20
Magnification: ×98,000 (A); ×38,300 (B)
Annulate Lamellae
Annulate lamellae are peculiar cytoplasmic organelles composed of stacked sheets of endoplasmic reticulum-like membranes interrupted by annuli or pores. They are most commonly found in oocytes as well as embryonic and neoplastic cells, but they can be observed, albeit to a lesser degree, in virtually any eukaryotic cell type including CHO cells as shown here.
Both the origin and the function(s) of annulate lamellae are poorly understood. In many cell types, annulate lamellae seem to originate from the nuclear envelope inasmuch as they are often apposed to it, as can be seen in the lower right corner of panel A. Here, a cross-sectioned single annulate lamellae with a pore (arrowhead) is located adjacent to the nuclear envelope with nuclear pore complexes (NPC). Thus, for embryonic cells, annulate lamellae have been proposed to transiently store or accumulate excess nuclear pore material and to be transitory structures. Other investigators have concluded that annulate lamellae are derived from the rough endoplasmic reticulum because they were found to be continuous with its cisternae, as can also be seen in panel A (RER). In panel A, a cross-sectioned annulate lamellae is depicted, which consists of lamellae that are arranged in a parallel array. The lamellae are interrupted by pores (arrowheads), which are aligned. The pores are spanned by a diaphragm-like structure and exhibit small peripheral granules. Continuity between single lamellae and cisternae of the rough endoplasmic reticulum (RER in A and B) is obvious. In panel B, a grazing section through an annulate lamellae is shown. Numerous pores (arrowhead) are seen in a face-on view, which reveals the presence of a large central granule and smaller annular granules in the pores. These are characteristic features of nuclear pore complexes. By morphological criteria, the pores of annulate lamellae are indistinguishable from nuclear pore complexes.
References
Beckhelling C, Chang P, Chevalier S, Ford C, Houliston E (2003) Pre-M phase-promoting factor associates with annulate lamellae in Xenopus oocytes and egg extracts. Mol Biol Cell 14:1125
Cordes VC, Reidenbach S, Franke WW (1995) High content of a nuclear pore complex protein in cytoplasmic annulate lamellae of Xenopus oocytes. Eur J Cell Biol 68:240
Cordes VC, Reidenbach S, Franke WW (1996) Cytoplasmic annulate lamellae in cultured cells: composition, distribution, and mitotic behavior. Cell Tissue Res 284:177
Franke WW, Scheer U, Fritsch H (1972) Intranuclear and cytoplasmic annulate lamellae in plant cells. J Cell Biol 53:823
Imreh G, Hallberg E (2000) An integral membrane protein from the nuclear pore complex is also present in the annulate lamellae: implications for annulate lamella formation. Exp Cell Res 259:180
Kessel RG (1992) Annulate lamellae: a last frontier in cellular organelles. Int Rev Cytol 133:43
Ralle T, Grund C, Franke WW, Stick R (2004) Intranuclear membrane structure formations by CaaX-containing nuclear proteins. J Cell Sci 117:6095
Scheer U, Franke WW (1969) Negative staining and adenosine triphosphatase activity of annulate lamellae of newt oocytes. J Cell Biol 42:519
Stafstrom JP, Staehelin LA (1984) Are annulate lamellae in the Drosophila embryo the result of overproduction of nuclear pore components? J Cell Biol 98:699
Sutovsky P, Simerly C, Hewitson L, Schatten G (1998) Assembly of nuclear pore complexes and annulate lamellae promotes normal pronuclear development in fertilized mammalian oocytes. J Cell Sci 111:2841


Fig. 21
Magnification: ×53,500 (A); ×57,800 (B)
Rough Endoplasmic Reticulum: Site of Protein Translocation and Initiation of Protein N-glycosylation
The membranes of the rough endoplasmic reticulum (RER) represent the site of co-translational vectorial transfer of de novo synthesized proteins from the cytosol to the ER and of lipid biosynthesis. After translocation, posttranslational protein modifications occur in the cisternal lumen of the endoplasmic reticulum.
In the rough endoplasmic reticulum (RER), soluble proteins become fully translocated into the cisternal lumen, whereas transmembrane proteins are translocated only partially and become inserted in the ER membrane to reside here or to become routed to other cellular destinations. The process of protein translocation is mediated by the translocon or Sec61 complex, which is a sophisticated modular protein machinery. Panel A depicts the immunogold localization of Sec61 protein at the RER and outer nuclear membrane in a rat liver hepatocyte. General structural and functional aspects of the translocon seem to be highly conserved among prokaryotic and eukaryotic organisms. Although proposed, the translocon seems not to function in the dislocation of misfolded glycoproteins from the RER to the cytosol. Based on high-resolution structural analyses, the translocon consists of a doughnut-like structure with a central hydrophilic pore as the most striking feature. The pore diameter in a ribosome bound translocon is 40–60 Å and that of a ribosome free translocon is 9–15 Å. A ribosome may be associated at the cytosolic side with its tunnel coaxially oriented over the Sec61 pore.
The oligosaccharyltransferase and the signal peptidase constitute major translocon-associated proteins not directly involved in the pore formation and in the translocation process. The oligosaccharyltransferase is a hetero-oligomeric complex composed of at least nine subunits showing significant homology from yeast to mammalian cells. For functional reasons it neighbors the translocon. It acts on nascent polypeptides while they are being translocated and catalyzes the en bloc transfer of a lipid-linked preassembled oligosaccharide (glucose3mannose9 N-acetylglucosamine2) to selected Asn-X-Ser/Thr sequences (X any amino acid except proline) of nascent polypeptide chains. This reaction occurs exclusively on the luminal side of the RER membrane and represents a key step in the pathway of protein N-glycosylation, which is an essential and highly conserved protein modification. The asparagine-linked oligosaccharide can be detected by the plant lectin Concanavalin A (Con A) because of its reactivity with oligosaccharides containing glucose or mannose. In panels B and C, Con A-gold labeling of the RER and of smooth endoplasmic reticulum (SER) of rat liver hepatocytes is shown. In regions containing SER, Con A labeling is also the result of the presence of glycogen. The lumen of the liver sinus and bile capillary (BC) are unlabeled.
References
Alder NN, Shen Y, Brodsky JL, Hendershot LM, Johnson AE (2005) The molecular mechanisms underlying BiP-mediated gating of the Sec61 translocon of the endoplasmic reticulum. J Cell Biol 168:389
Breitling J, Aebi M (2013) N-linked protein glycosylation in the endoplasmic reticulum. Cold Spring Harb Perspect Biol 3:a013359
Chavan M, Lennarz W (2006) The molecular basis of coupling of translocation and N-glycosylation. Trends Biochem Sci 31:17
Frauenfeld J et al (2011) Cryo-EM structure of the ribosome SecYE complex in the membrane environment. Nat Struct Mol Biol 18:614
Johnson AE (2009) The structural and functional coupling of two molecular machines, the ribosome and the translocon. J Cell Biol 185:765
Kelleher DJ, Gilmore R (2006) An evolving view of the eukaryotic oligosaccharyltransferase. Glycobiology 16:47R
Mandon EC, Trueman SF, Gilmore R (2013) Protein translocation across the rough endoplasmic reticulum. Cold Spring Harb Perspect Biol 2:a013342
Menetret JF, Hegde RS, Heinrich SU, Chandramouli P, Ludtke SJ, Rapoport TA, Akey CW (2005) Architecture of the ribosome-channel complex derived from native membranes. J Mol Biol 348:445
Osborne AR, Rapoport TA, van den Berg B (2005) Protein translocation by the Sec61/SecY channel. Annu Rev Cell Dev Biol 21:529
Zuber C, Roth J (2009) N-Glycosylation. In: Gabius H-J (ed) The sugar code. Fundamentals of glycosciences. Wiley/VCH, Weinheim, p 87


Fig. 22
Magnification: ×71,500 (A); ×11,000 (B); ×35,500 (C)
Oligosaccharide Trimming, Reglucosylation, and Protein Quality Control in the Rough Endoplasmic Reticulum
In the rough endoplasmic reticulum, first modifications on asparagine-linked oligosaccharides occur and include the removal of all three glucose residues by α-glucosidase I (Gls I) and II (Gls II) and of some mannose residues by ER α-mannosidases I (ER Man I) and II (ER Man II), as illustrated in the scheme. These trimming reactions and the resulting specific oligosaccharides are important for protein quality control. Proper folding and correct assembly of glycoproteins are constantly monitored by a quality control machinery composed of chaperones, lectins such as calnexin and calreticulin, glucosidase II and UDP-glucose:glycoprotein glucosyltransferase (for additional details, cf. Fig. 33). Conversely, defective oligosaccharide trimming has been recognized as the cause of congenital disorders of glycosylation.


In panel A, immunogold labeling demonstrates trimming glucosidase II in the nuclear envelope (arrowheads) and the lumen of endoplasmic reticulum cisternae. Both glucosidase I and II are present in the same endoplasmic reticulum cisternae as shown by double immunogold labeling with large gold particles for the detection of glucosidase I and small particles for glucosidase II (inset in panel A). The importance of the trimming of the outer glucose residue by glucosidase I is unknown, but that of the second glucose by glucosidase II provides a positive signal for protein folding. Glucosidase II also functions in the dissociation of complexes between calnexin/calreticulin and glycoproteins bearing monoglucosylated oligosaccharides.
Panel B shows glucosyltransferase immunolabeling in nuclear envelope (arrowheads) and the cisternal lumen of rough endoplasmic reticulum in a distribution similar to glucosidase II. Glucosyltransferase is unique in that it serves in a two-fold function as a protein folding sensor and as a glycosyltransferase. The enzyme recognizes incorrectly folded glycoproteins and, by reglucosylating their oligosaccharides, tags the glycoprotein for entry in another calnexin/calreticulin cycle.
References
Braakman I, Bulleid NJ (2011) Protein folding and modification in the mammalian endoplasmic reticulum. Annu Rev Biochem 80:71
Braakman I, Hebert DN (2013) Protein folding in the endoplasmic reticulum. Cold Spring Harb Perspect Biol 3:a013169
Freeze HH (2006) Genetic defects in the human glycome. Nat Rev Genet 7:537
Hennet T (2009) Diseases of glycosylation. In: Gabius HJ (ed) The sugar code. Fundamentals of glycosciences. Wiley-Blackwell, Weinheim, p 365
Parodi AJ (2000) Protein glucosylation and its role in protein folding. Annu Rev Biochem 69:69
Roth J et al (2010) Protein N-glycosylation, protein folding, and protein quality control. Mol Cells 30:497
Ruggiano A, Foresti O, Carvhalo P (2014) ER-associated degradation: protein quality control and beyond. J Cell Biol 204:869
Smith MH, Ploegh HL, Weissman JS (2011) Road to ruin: targeting proteins for degradation in the endoplasmic reticulum. Science 334:1086
Vembar SS, Brodsky JL (2008) One step at a time: endoplasmic reticulum-associated degradation. Nat Rev Mol Cell Biol 9:944
Zuber C, Roth J (2009) N-Glycosylation. In: Gabius H-J (ed) The sugar code. Fundamentals of glycosciences. Wiley/VCH, Weinheim, p 87


Fig. 23
Magnification: ×53,000 (A); inset ×70,300; ×48,000 (B)
Rough Endoplasmic Reticulum: Storage Site of Aggregates of Misfolded Glycoproteins
Protein misfolding occurs naturally at a high rate, or as a consequence of mutations and under various forms of cellular stress such as hyperthermia, hypoxia, starvation, experimental inhibition of protein synthesis by puromycin or of N-glycosylation by tunicamycin, and inhibition of disulfide bridge formation by reducing agents. Accumulation of misfolded proteins also occurs in congenital human protein folding diseases, e.g., cystic fibrosis, α-1-antitrypsin deficiency, and congenital goitrous hypothyreoidism.
The fate of misfolded proteins can be quite diverse. In the best case scenario, they will be efficiently and completely dislocated to the cytoplasm and degraded by the ubiquitin-proteasome system without harmful consequences for the cells, and without morphological changes of the rough endoplasmic reticulum. Under unfavorable conditions, they may aggregate in the lumen of the rough endoplasmic reticulum. The classical manifestation of this form of protein aggregation is the Mott cells, which represent plasma cells with stored immunoglobulins. This and other types of protein inclusions are generally called Russell bodies, which can also be observed in secretory cells other than plasma cells. Because such protein aggregates cannot be dislocated to the cytosol, the cisternal space of the entire rough endoplasmic reticulum of Mott cells may become greatly distended (asterisks in panel A). Local protein aggregates represented by the intracisternal granules in the lumen of the rough endoplasmic reticulum can occur under conditions of starvation or treatment with puromycin aminonucleoside. In panel B, part of a pancreatic acinar cell with intracisternal granules and a zymogen granule (ZG), both with immunogold labeling for amylase, is shown.
The functional consequences of protein accumulation in the ER are many-fold and principally result in the induction of the unfolded protein response. The unfolded protein response pathway, which involves Ire1α, PERK, and ATF6, controls the expression of various ER chaperones but may also induce apoptosis and inflammation and causes translational attenuation. This signaling pathway is highly conserved from yeast to mammalian cells.
References
Alanen A, Pira U, Lassila O, Roth J, Franklin RM (1985) Mott cells are plasma cells defective in immunoglobulin secretion. Eur J Immunol 15:235
Jonikas MC et al (2009) Comprehensive characterization of genes required for protein folding in the endoplasmic reticulum. Science 323:1693
Kim PS, Arvan P (1998) Endocrinopathies in the family of endoplasmic reticulum (ER) storage diseases: disorders of protein trafficking and the role of ER molecular chaperones. Endocr Rev 19:173
Kopito RR (2000) Aggresomes, inclusion bodies and protein aggregation. Trends Cell Biol 10:524
Ozcan L, Tabas I (2012) Role of endoplasmic reticulum stress in metabolic disease and other disorders. Annu Rev Med 63:317
Palade G (1956) Intracisternal granules in the exocrine cells of the pancreas. J Biophys Biochem Cytol 2:417
Schröder M, Kaufman RJ (2005) The mammalian unfolded protein response. Annu Rev Biochem 74:739
Tooze J, Kern HF, Fuller SD, Howell KE (1989) Condensation-sorting events in the rough endoplasmic reticulum of exocrine pancreatic cells. J Cell Biol 109:35
Valetti C, Grossi C, Milstein C, Sitia R (1991) Russel bodies: a general response of secretory cells to synthesis of mutant immunoglobulin which can neither exit from, nor be degraded in the endoplasmic reticulum. J Cell Biol 115:983
Walter P, Ron D (2011) The unfolded protein response: from stress pathway to homeostatic regulation. Science 334:1081
Yam GHF, Gaplovska-Kysela K, Zuber C, Roth J (2007) Aggregated myocilin induces Russell bodies and causes apoptosis – implications for the pathogenesis of myocilin-caused primary open-angle glaucoma. Am J Pathol 170:100


Fig. 24
Magnification: ×20,500 (A); ×95,000 (B)
Plasma Cell Endoplasmic Reticulum: Storage Site of Excess Immunoglobulins
Plasma cells are highly active secretory cells that secrete large amounts of the polymeric form of IgM. They originate from B lymphocytes that undergo impressive developmental changes upon contact with antigens. Morphologically, this is characterized by the formation of a highly complex network of rough endoplasmic reticulum cisternae, as shown in panel A, which strongly contrasts the scarce number of rough endoplasmic reticulum cisternae of B lymphocytes (cf. Fig. 194).
De novo synthesized Ig-μ chains assemble with L chains to form μL and μ2L2 subunits that polymerize into secretion-competent IgM polymers. During this process, interaction with various machinery proteins of the ER protein quality control such as BiP, protein disulfide isomerase, and calreticulin as well the pGI protein ERGIC-53 occurs to promote assembly and polymerization. However, unassembled and nonpolymerized subunits may occur that are retained in the endoplasmic reticulum and subjected to ERAD (cf. Figs. 23 and 24). Both the differentiation-associated strong increase in immunoglobulin synthesis and the presence of nonpolymerized assembly intermediates has an impact on the unfolded protein response of plasma cells. Besides morphologically unaffected plasma cells with endoplasmic reticulum cisternae with a narrow lumen, as shown in panel A, others exhibit rough endoplasmic reticulum cisternae, all of which have a greatly distended lumen filled with an electron-dense material, an indication of protein accumulation (cf. also Fig. 24). Panel B shows a detail of the peripheral cytoplasm of two neighboring plasma cells (the arrowhead points to their plasma membranes) occupied with endoplasmic reticulum cisternae either presenting a narrow or a greatly distended lumen (asterisks). Although morphologically almost identical, this is causally different from the situation of mutant Ig-μ chains, which accumulate in the form of detergent-insoluble protein aggregates to form Russell bodies in Mott cells (cf. Fig. 24). Here, the surplus of unassembled and nonpolymerized Ig subunits most probably has resulted in exhaustion of the capacity of ERAD. As shown in panels C and D, even crystalline inclusions (asterisks) exist in the lumen of several rough endoplasmic reticulum cisternae, whereas adjacent cisternae exhibit only a slightly distended lumen. Of note, the drastic change of rough endoplasmic cisternae due to the crystalline inclusions has not resulted in the disruption of the ER membrane nor in dissociation of ribosomes. Although such protein accumulations by definition will cause ER stress, plasma cells, as shown here, exhibit no morphological signs of cellular damage such as apoptosis (cf. Fig. 15). Moreover, it is known that Mott cells remain viable.
References
Alanen A, Pira U, Lassila O, Roth J, Franklin RM (1985) Mott cells are plasma cells defective in immunoglobulin secretion. Eur J Immunol 15:235
Anelli T, Ceppi S, Bergamelli L, Cortini M, Masciarelli S, Valetti C, Sitia R (2007) Sequential steps and checkpoints in the early exocytic compartment during secretory IgM biogenesis. EMBO J 26:4177
Braakman I, Hebert DN (2013) Protein folding in the endoplasmic reticulum. Cold Spring Harb Perspect Biol 3:a013169
Gunn KE, Gifford NM, Mori K, Brewer JW (2004) A role for the unfolded protein response in optimizing antibody secretion. Mol Immunol 41:919
Kopito RR (2000) Aggresomes, inclusion bodies and protein aggregation. Trends Cell Biol 10:524
Mattioloi L, Anelli T, Fagioli C, Tacchetti C, Sitia R, Valetti C (2006) ER storage diseases: a role for ERGIC-53 in controlling the formation and shape of Russell bodies. J Cell Sci 119:2532
Reddy PM, Corley AB (1999) The contribution of ER quality control to the biologic functions of secretory IgM. Immunol Today 20:582
Sitia R, Neuberger M, Alberine C, Williams G, Milstein C (1990) Developmental regulation of IgM secretion: the role of the carboxy-terminal cysteine. Cell 60:781
Tagliavacca L, Anelli T, Fagioli C, Mezghrani A, Ruffato E, Sitia R (2003) The making of a professional secretory cell: architectural and functional changes in the ER during B lymphocyte plasma cell differentiation. Biol Chem 384:1273
Valetti C, Grossi C, Milstein C, Sitia R (1991) Russel bodies: a general response of secretory cells to synthesis of mutant immunoglobulin which can neither exit from, nor be degraded in the endoplasmic reticulum. J Cell Biol 115:983


Fig. 25
Magnification: ×2,500 (A); ×30,000 (B); ×22,500 (C); ×62,500 (D)
Russell Bodies and Aggresomes are Different Types of Protein Inclusion Bodies
It has already been mentioned that Russell bodies result from the aggregation of misfolded glycoproteins in the lumen of the rough endoplasmic reticulum. A giant Russell body similar in size to the nucleus is seen in panel A with adjacent normal appearing rough endoplasmic reticulum cisternae (RER), mitochondria (M), and lysosomes (Ly). A main structural feature of Russel bodies is their with ribosomes covered limiting membrane, the rough endoplasmic reticulum membrane.
Aggresomes, shown in panel B, are another type of inclusion body that results from the production of misfolded (glyco)proteins. They differ from Russell bodies in that the proteinaceous aggregates, which can be observed in the electron microscope as amorphous dense material (asterisk), are located in the cytosol and have no limiting membrane. This topographical difference is the result of the dislocation of the misfolded glycoproteins to the cytosol, where they eventually form aggregates. Furthermore, aggresomes, in contrast to Russell bodies, are composed not only of aggregates of ubiquitinated proteins but also contain proteasomes, selective autophagy receptors such as sequestosome1/p62, and chaperones. Fully formed aggresomes are surrounded by a cage of intermediate filaments of the vimentin type (IF) and are found close to the microtubule organizing center. They are formed from smaller cytosolic protein aggregates present throughout the cytosol by active minus-end directed transport along microtubules (MT). Aggresomes can be experimentally induced by the inhibition of proteasome activity or occur when the proteolytic capacity of the proteasomes is exhausted by the presence of aggregation-prone misfolded proteins. There is also evidence that protein aggregates can directly impair the proteasome activity.
Despite these differences, both Russell bodies and aggresomes are morphological manifestations of cellular indigestion often observed under various diseased states. In particular, inclusion bodies are commonly found in association with chronic neurodegenerative diseases such as Alzheimer’s and Parkinson’s disease and familial amyotrophic lateral sclerosis.
References
Alanen A, Pira U, Lassila O, Roth J, Franklin R (1985) Mott cells are plasma cells defective in immunoglobulin secretion. Eur J Immunol 15:235
Bence NF, Sampat RM, Kopito RR (2001) Impairment of the ubiquitin-proteasome system by protein aggregation. Science 292:1552
Garcia-Mata R, Bebök Z, Sorscher EJ, Sztul ES (1999) Characterization and dynamics of aggresome formation by a cytosolic GFP-chimera. J Cell Biol 146:1239
Johnston JA, Ward CL, Kopito RR (1998) Aggresomes: a cellular response to misfolded proteins. J Cell Biol 143:1883
Kawaguchi Y, Kovacs JJ, McLaurin A, Vance JM, Ito A, Yao TP (2003) The deacetylase HDAC6 regulates aggresome formation and cell viability in response to misfolded protein stress. Cell 115:727
Kopito RR (2000) Aggresomes, inclusion bodies and protein aggregation. Trends Cell Biol 10:524
Kopito RR, Sitia R (2000) Aggresomes and Russell bodies – symptoms of cellular indigestion? EMBO Rep 1:225
Seibenhener ML, Babu JR, Geetha T, Wong HC, Krishna NR, Wooten MW (2004) Sequestosome 1/p62 is a polyubiquitin chain binding protein involved in ubiquitin proteasome degradation. Mol Cell Biol 24:8055
Valetti C, Grossi C, Milstein C, Sitia R (1991) Russell bodies: a general response of secretory cells to synthesis of mutant immunoglobulin which can neither exit from, nor be degraded in, the endoplasmic reticulum. J Cell Biol 115:983
Yam GHF, Gaplovska-Kysela K, Zuber C, Roth J (2007) Aggregated myocilin induces Russell bodies and causes apoptosis – implications for the pathogenesis of myocilin-caused primary open-angle glaucoma. Am J Pathol 170:100


Fig. 26
Magnification: ×19,000 (A); ×48,000 (B)
Smooth Endoplasmic Reticulum
Lacking attached ribosomes, the smooth endoplasmic reticulum (SER) forms a complex membranous system that is linked to various cellular functions. The main SER tasks include synthesis of lipids, maintenance of calcium homeostasis, and detoxification reactions necessary for the conversion of harmful water-insoluble substances into water-soluble compounds that are more suitable for excretion through the kidney. Specialized SER regions are the membraneous networks and the terminal cisterns of the sarcoplasmic reticulum (cf. Fig. 170). Although continuous with the rough endoplasmic reticulum, the SER is organized in a different way. The differences are perfectly discernible in hepatocytes as shown in panel A.
The rough endoplasmic reticulum (RER) visible in the upper left corner of the micrograph forms regular stacks of flat cisternae in parallel orientation, whereas the SER consists of interconnected tubular and finger-like architectures that appear tangled up, forming membranous convolutions; ER shaping proteins, such as reticulons, play pivotal roles in the formation of the characteristic SER and RER- morphologies. In hepatocytes and in steroid hormone-producing cells, extended SER membrane convolutes occupy wide areas of the cytoplasm. In the electron microscope, myriads of smooth ER membrane profiles characterize these cytoplasmic areas. There is a close spatial relationship of SER membranes and glycogen particles (asterisk), which may reflect functional connections underlined by enzymes of the glycogen metabolism that were found associated with SER membranes.
Membrane continuities shown in detail in panel B (arrows) reflect the close functional cooperations and common tasks of smooth and rough endoplasmic reticulum. Synthesis of the different components of lipoproteins and formation of lipoprotein particles is one example, which also can be shown morphologically. Very low density lipoprotein particles (VLDLs) produced by the hepatocytes and secreted into the space of Disse (cf. Figs. 124 and 125) are visible as electron-dense globules within the SER lumina (arrows). They are particularly frequent close to the SER-RER-transitions.
Under physiological conditions, de novo synthesis of ER-membranes and removal are in balance and export out of the ER is accompanied by input via recycling membrane constituents. The overall size of the endoplasmic reticulum is thought to remain constant, but ER-membranes and compartments are highly dynamic and change their shapes continuously. Sizes and shapes of ER-compartments are determined by opposing forces of assembly connected with membrane fusion and disassembly connected with membrane fragmentation.
For ER-organization, the microenvironments and the cytoskeleton, especially the actin filament system and microtubules, have crucial roles. Differences in the appearance of the cytoplasmic “matrices” of RER and SER are clearly discernible in the electron microscope. RER cisternae visible in the upper left quarter of the micrograph are embedded in dense, compact “matrices” that are lacking in the SER cytoplasmic regions (cf. also Figs. 124 and 125).
M: mitochondria; PO: peroxisome
References
Baumann O, Walz B (2001) Endoplasmic reticulum of animal cells and its organization into structural and functional domains. Int Rev Cytol 205:149
Chen S, Novick P, Ferro-Novick S (2013) ER structure and function. Curr Opin Cell Biol 25:428
English AR, Zurek N, Voeltz GK (2009) Peripheral ER structure and function. Curr Opin Cell Biol 21:596
Iinuma T, Aoki T, Arasaki K, Hirose H, Yamamoto A, Samata R, Hauri H-P, Arimitsu N, Tagaya M, Tani K (2009) Role of syntaxin 18 in the organization of the endoplasmic reticulum subdomains. J Cell Sci 122:1680
Joensuu M, Belevich I, Rämö O, Nevzorov I, Vihinen H, Puhka M, Witkos TM, Lowe M, Vartiainen MK, Jokitalo E (2014) ER sheet persistence is coupled to myosin 1c-regulated dynamic actin filament arrays. Mol Biol Cell 25:1111
Lavoie C, Paiement J (2008) Topology of molecular machines of the endoplasmic reticulum: a compilation of proteomics and cytological data. Histochem Cell Biol 129:117
Paiement J, Bergeron J (2001) The shape of things to come: regulation of shape changes in endoplasmic reticulum. Biochem Cell Biol 79:587
Park SH, Blackstone C (2010) Further assembly required: construction and dynamics of the endoplasmic reticulum network. EMBO Rep 11:515
Shibata Y, Shemesh T, Prinz WA, Palazzo AF, Kozlov MM, Rapoport TA (2010) Mechanisms determining the morphology of the peripheral ER. Cell 143:774
Steegmaier M, Oorschot V, Klumperman J, Scheller RH (2000) Syntaxin 17 is abundant in steroidogenic cells and implicated in smooth endoplasmic reticulum membrane dynamics. Mol Biol Cell 11:2719
Van Meer G, Voelker DR, Feigenson GW (2008) Membrane lipids: where they are and how they behave. Nat Rev Mol Cell Biol 9:112


Fig. 27
Magnification: ×32,800 (A), ×55,000 (B)
Proliferation of the Smooth Endoplasmic Reticulum
Hepatocytes and steroid-producing cells contain abundant smooth endoplasmic reticulum in contrast to most other cell types. The smooth endoplasmic reticulum membranes of liver hepatocytes amount to 16 % of total cellular membranes, whereas in pancreatic exocrine cells this value is less than 1 %. The amount of smooth endoplasmic reticulum can increase rapidly and reversibly depending on functional demands, and its phenobarbital-induced proliferation represents a classical example for such a situation. After withdrawal of the drug, the excess smooth endoplasmic reticulum is removed by autophagy (cf. Figs. 74, 75, and 76 for aspects of autophagy).
In hereditary disorders of bilirubin metabolism resulting in predominantly unconjugated hyperbilirubinemia, the cytoplasm of liver hepatocytes can be filled with smooth endoplasmic reticulum (SER) at the expense of the rough endoplasmic reticulum and contains depositions of bilirubin (arrows and inset). These disorders include the Crigler-Najjar syndromes I and II and the Gilbert syndrome. The electron micrograph shows hepatocytes from a liver biopsy of a patient with Crigler-Najjar syndrome. Crigler-Najjar syndrome is inherited in an autosomal recessive mode. The molecular defect lies in the hepatic bilirubin UDP-glucoronosyltransferase A1 isoform, whose gene locus is located on chromosome 2q37. Genetic aberrations can occur in any of its five exons in the form of deletions, insertions, missense mutations, and premature stop codons. As a consequence, hepatic bilirubin UDP-glucoronosyltransferase activity can be undetectable or reduced and non-hemolytic icterus caused by increased serum concentrations of unconjugated bilirubin occurs during the first days of life. When untreated, fatal kernicterus causing bilirubin encephalopathy results. However, long-term survival could be achieved by orthotopic liver transplantation.
References
Bosma P et al (1992a) Mechanism of inherited deficiencies of multiple UDP-glucoronosyltransferase isoforms in two patients with Crigler-Najjar syndrome, type I. FASEB J 6:2859
Bosma P et al (1992b) Sequence of exons and the flanking regions of human bilirubin-UPD-glucoronosyltransferase gene complex and identification of a genetic mutation in a patient with Crigler-Najjar syndrome, type I. Hepatology 15:941
Bosma P et al (1994) Bilirubin UDP-glucoronosyltransferase I is the only relevant bilirubin glucoronidating isoform in man. J Biol Chem 269:17960
Crigler J, Najjar V (1952) Congenital familial non-hemolytic jaundice with kernicterus. Pediatrics 10:169
Roy Chowdhury J, Wolkoff A, Roy Chowdhury N, Arias I (2001) Hereditary jaundice and disorders of bilirubin metabolism. In: The metabolic & molecular bases of inherited diseases. McGraw-Hill, New York, p 3063


Fig. 28
Magnification: ×9,600; ×13,000 (inset)
Transitional Elements of Rough Endoplasmic Reticulum and Pre-Golgi Intermediates
The interface between the rough endoplasmic reticulum and the Golgi apparatus consists of a complex and highly dynamic structure, the pre-Golgi intermediates (pGI). They are also referred to as intermediate (or salvage) compartment, ERGIC-53, or vesicular-tubular clusters (VTC) and represent intermediates for transport out of the endoplasmic reticulum or for recycling to the endoplasmic reticulum.
The exit of cargo from the endoplasmic reticulum occurs at specialized, morphologically defined sites, the transitional elements of the rough endoplasmic reticulum that are partly devoid of ribosomes (TE in panels A and C) and exhibit coated buds (arrowheads in panels A and C), which give rise to vesicles. Coated buds exist also at the outer nuclear membrane (arrowheads in panels E1 and E2) and apparently give rise to vesicles located in the narrow space between nuclear envelope and Golgi apparatus (panels E1 and E2). In addition to coated buds, tubular extensions exist at the transitional elements (arrowheads in panel D). Located between the transitional elements and the cis side of the Golgi apparatus are clusters of vesicles and tubules, the pGI (pGI in panels A, C and D). pGI together with transitional elements of rough endoplasmic reticulum exist also distant from the Golgi apparatus in the peripheral cytoplasm (panels C and D) and move from there along microtubules to the Golgi apparatus. Transitional elements and pGI are not only constituents of secretory cells (panel A shows part of an endocrine B-cell with secretory granules – SG) but also of nonsecretory cells such as Chinese hamster ovary (CHO) cells (panel C) or HepG2 hepatoma cells (panels D and E). The pGI are enriched in ERGIC-53 or p58 (rat homologue of human ERGIC-53), providing a marker for their identification by immunolabeling (panel B).


Intense studies have unraveled many aspects of the molecular mechanism of cargo selection in the transitional elements of the rough endoplasmic reticulum and ER-to-Golgi transport and recycling. Cytosolic coat proteins (COP) such as Sar1, Sec23/Sec24, and Sec13/Sec31 are recruited to transitional elements, resulting in the formation of COPII-coated buds (see diagram) into which cargo is actively sorted. COPII-coated vesicles then carry out the anterograde transport. Such vesicles lose their coat and fuse with each other or neighboring vesicular-tubular clusters. There is also evidence for the direct en bloc formation of large pleomorphic and tubular carriers from the transitional elements of the rough endoplasmic reticulum (cf. Figs. 30 and 31). pGI are not only involved in anterograde COPII-mediated transport but also in retrograde transport, which is mediated by the COPI vesicular pathway. The COPI and COPII coats are morphologically distinct from the clathrin coats of buds and vesicles at the trans side of the Golgi apparatus or the plasma membrane.
References
Appenzeller-Herzog C, Hauri HP (2006) The ER-Golgi intermediate compartment (ERGIC): in search of its identity and function. J Cell Sci 119:2173
Bannykh SI, Balch WE (1997) Membrane dynamics at the endoplasmic reticulum Golgi interface. J Cell Biol 138:1
Brandizzi F, Barlowe C (2013) Organization of the ER-Golgi interface for membrane traffic control. Nat Rev Mol Cell Biol 14: 382
Fan JY, Roth J, Zuber C (2003) Ultrastructural analysis of transitional endoplasmic reticulum and pre-Golgi intermediates: a highway for cars and trucks. Histochem Cell Biol 120:455
Gurkan C, Stagg SM, LaPointe P, Balch WE (2006) The COPII cage: unifying principles of vesicle coat assembly. Nat Rev Mol Cell Biol 7:727
Hammond AT, Glick BS (2000) Dynamics of transitional endoplasmic reticulum sites in vertebrate cells. Mol Biol Cell 11:3013
Liu W, Lippincott-Schwartz J (2005) Illuminating COPII coat dynamics. Nat Struct Mol Biol 12:106
Saraste J, Palade GE, Farquhar MG (1987) Antibodies to rat pancreas Golgi subfractions: identification of a 58-kD cis-Golgi protein. J Cell Biol 105:2021
Schweizer A, Fransen JAM, Bächi T, Ginsel L, Hauri HP (1988) Identification, by a monoclonal antibody, of a 53-kD protein associated with a tubulo-vesicular compartment at the cis-side of the Golgi apparatus. J Cell Biol 107:1643
Stagg SM, LaPointe P, Razvi A, Gurkan C, Potter CS, Carragher B, Balch WE (2008) Structural basis for cargo regulation of COPII coat assembly. Cell 134:474
Zanetti G, Pahuja KB, Studer S, Shim S, Schekman R (2012) COPII and the regulation of protein sorting in mammals. Nat Cell Biol 14:20
Zeuschner D, Geerts WJC, vanDonselaar E, Humbel BM, Slot JW, Koster AJ, Klumperman J (2006) Immuno-electron tomography of ER exit sites reveals the existence of free COPII-coated transport carriers. Nat Cell Biol 8:377


Fig. 29
Magnification: ×50,000 (A); ×66,500 (B); ×74,000 (C); ×73,000 (D); ×90,000 (E)
Golgi Apparatus-Associated Pre-Golgi Intermediates: Details in Series
The pre-Golgi intermediates (pGI) are usually portrayed as being situated between the transitional elements of the endoplasmic reticulum and the cis side of the Golgi apparatus. This standard situation for Golgi apparatus-associated pGI is frequently encountered in single ultrathin sections and can also be found by serial section analysis. From morphometric analysis of reconstructions of rat basophilic leukemia RBL 2H3 cells, Golgi apparatus-associated pGI have an average diameter of 0.4 μm and were composed of 35 vesicles on average. There is, however, quite some variation in the standard location of Golgi apparatus-associated pGI. They can be located in a narrow space between the outer nuclear membrane and the Golgi apparatus (cf. Fig. 29). Another variation is seen in the 12 consecutive serial sections from CHO cells shown here. Rough endoplasmic reticulum cisternae (ER) can be followed as they develop in transitional elements (sections 3–10) that exhibit coated buds. Likewise, pGI composed of vesicles and tubules of varying length can be unequivocally identified (asterisk in sections 4–10). It is worth noting that in most of the serial sections, transitional elements and pGI face and twist around the lateral aspect and not the cis side of a Golgi apparatus cisternal stack (G). This situation is particularly obvious in the consecutive serial sections 6–9. Only in serial section 10 does the pGI partially face the cis side of the Golgi apparatus stack. Morphometric analysis of serial sections of pGI from the CHO cells revealed an average size of 0.7 μm. A similar value was estimated for the size of pGI in mouse pancreatic beta cells. Based on measurements of serial sections, tubules of Golgi apparatus-associated pGI in pancreatic beta cells had an average diameter of 115 nm (range of 60–195 nm) and a length up to 500 nm. Altogether, these data demonstrate the great variability in the positioning of Golgi apparatus-associated pGI.
Figure from Fan et al. (2007) Histochem Cell Biol 128: 161.
References
Appenzeller-Herzog C, Hauri HP (2006) The ER-Golgi intermediate compartment (ERGIC): in search of its identity and function. J Cell Sci 119:2173
Bannykh SI, Balch WE (1997) Membrane dynamics at the endoplasmic reticulum Golgi interface. J Cell Biol 138:1
Fan JY, Roth J, Zuber C (2003) Ultrastructural analysis of transitional endoplasmic reticulum and pre-Golgi intermediates: a highway for cars and trucks. Histochem Cell Biol 120:455
Fan JY, Roth J, Zuber C (2007) Expression of mutant Ins2C96Y results in enhanced tubule formation causing enlargement of pre-Golgi intermediates in CHO cells. Histochem Cell Biol 128:161
Gurkan C, Stagg SM, LaPointe P, Balch WE (2006) The COPII cage: unifying principles of vesicle coat assembly. Nat Rev Mol Cell Biol 7:727
Hammond AT, Glick BS (2000) Dynamics of transitional endoplasmic reticulum sites in vertebrate cells. Mol Biol Cell 11:3013
Lee MC, Miller EA, Goldberg J, Orci L, Schekman R (2004) Bi-directional protein transport between the ER and Golgi. Annu Rev Cell Dev Biol 20:87
Liu W, Lippincott-Schwartz J (2005) Illuminating COPII coat dynamics. Nat Struct Mol Biol 12:106
Simpson JC, Nilsson T, Pepperkok R (2006) Biogenesis of tubular ER-to-Golgi transport intermediates. Mol Biol Cell 17:723
Stagg SM, LaPointe P, Razvi A, Gurkan C, Potter CS, Carragher B, Balch WE (2008) Structural basis for cargo regulation of COPII coat assembly. Cell 134:474
Verissimo F, Pepperkok R (2013) Imaging ER-to-Golgi transport: towards a systems view. J Cell Sci 22:5091
Zanetti G, Pahuja KB, Studer S, Shim S, Schekman R (2012) COPII and the regulation of protein sorting in mammals. Nat Cell Biol 14:20
Zeuschner D, Geerts WJC, vanDonselaar E, Humbel BM, Slot JW, Koster AJ, Klumperman J (2006) Immuno-electron tomography of ER exit sites reveals the existence of free COPII-coated transport carriers. Nat Cell Biol 8:377


Fig. 30
Magnification: ×30,500
Peripheral Pre-Golgi Intermediates: Size Matters
Pre-Golgi intermediates (pGI) exist in two different locations: associated with the Golgi apparatus (cf. Fig. 30) and in peripheral sites distant from the Golgi apparatus. Both types of pGI have a similar fine structure and size. Peripheral pGI function in cargo traffic to the Golgi apparatus as well, and, for this to occur, are transported to the Golgi apparatus in a microtubule-dependent fashion.
Peripheral pGI can be easily missed in single ultrathin sections, and their structural complexity can be best appreciated by serial section analysis. Panel A shows eight consecutive serial sections, which encompass an entire peripheral pGI of a CHO cell. Different appearances of a twisting rough endoplasmic reticulum cisterna (ER) can be seen in all serial sections, which gives rise to a transitional element exhibiting buds (section 2 and arrow in section 4). The pGI itself consists of a cluster of vesicular profiles and many straight or slightly curved tubules (outlined by a broken line in sections 2–7). In panel B, three consecutive serial sections out of a larger series are shown and illustrate the structural complexity of transitional elements and a pGI from pancreatic beta cells. A transitional element (TE) of the rough endoplasmic reticulum can be seen in all three consecutive serial sections. In section B2, it exhibits a canonical coated bud (arrow), while in section B1 it forms a long tubular structure without a recognizable coat (arrowheads). In section B3, another transitional element shows two distinct constrictions (arrowheads). The distal one results in a distended portion without a recognizable coat (asterisk). Next to it, a large vesicular profile is present (asterisk). The pGI as seen in the three consecutive serial sections is composed of vesicular structures of greatly varying size and tubules of a mean diameter of 115 nm and up to 500 nm long.
The existence of tubular extensions and large pleomorphic structures at transitional elements and of long tubules as well as vesicular and pleomorphic structures larger in size than the 60–80 nm COPII vesicles is related to a long-standing puzzle, namely how large-size cargo exits transitional elements and is transported to the Golgi apparatus. The 900 kDa procollagen VII trimer with a ≈ 300 nm rod-like structure exceeds the size of COPII buds and vesicles. A breakthrough for the field was the discovery that TANGO 1, cTAGE5, and Sedlin jointly act in procollagen VII ER export. It has been proposed that TANGO1 interaction with Sec23/24 interferes with the completion of the COPII coat formation and allows loading of procollagen VII in the growing ER bud. Upon completion of cargo loading, TANGO1 dissociates and permits completion of the COPII coat by Sec13/31. Both TANGO1 and cTAGE5 can interact with Sec23/24 and form a complex through interaction of their coiled-coil motifs. Therefore, cTAGE5 seems to function as a TANGO1 coreceptor. Sedlin, on the other hand, is recruited by TANGO1 to COPII buds and binds Sar1 for efficient cycling to prevent membrane constriction and vesicle formation before the precollagen VII fibers are completely moved in the buds. In agreement, mutations of the SEDL gene cause spondyloepiphyseal dysplasia tarda, which is characterized by defects in chondrogenesis resulting from impaired secretion of extracellular matrix components including precollagen II.
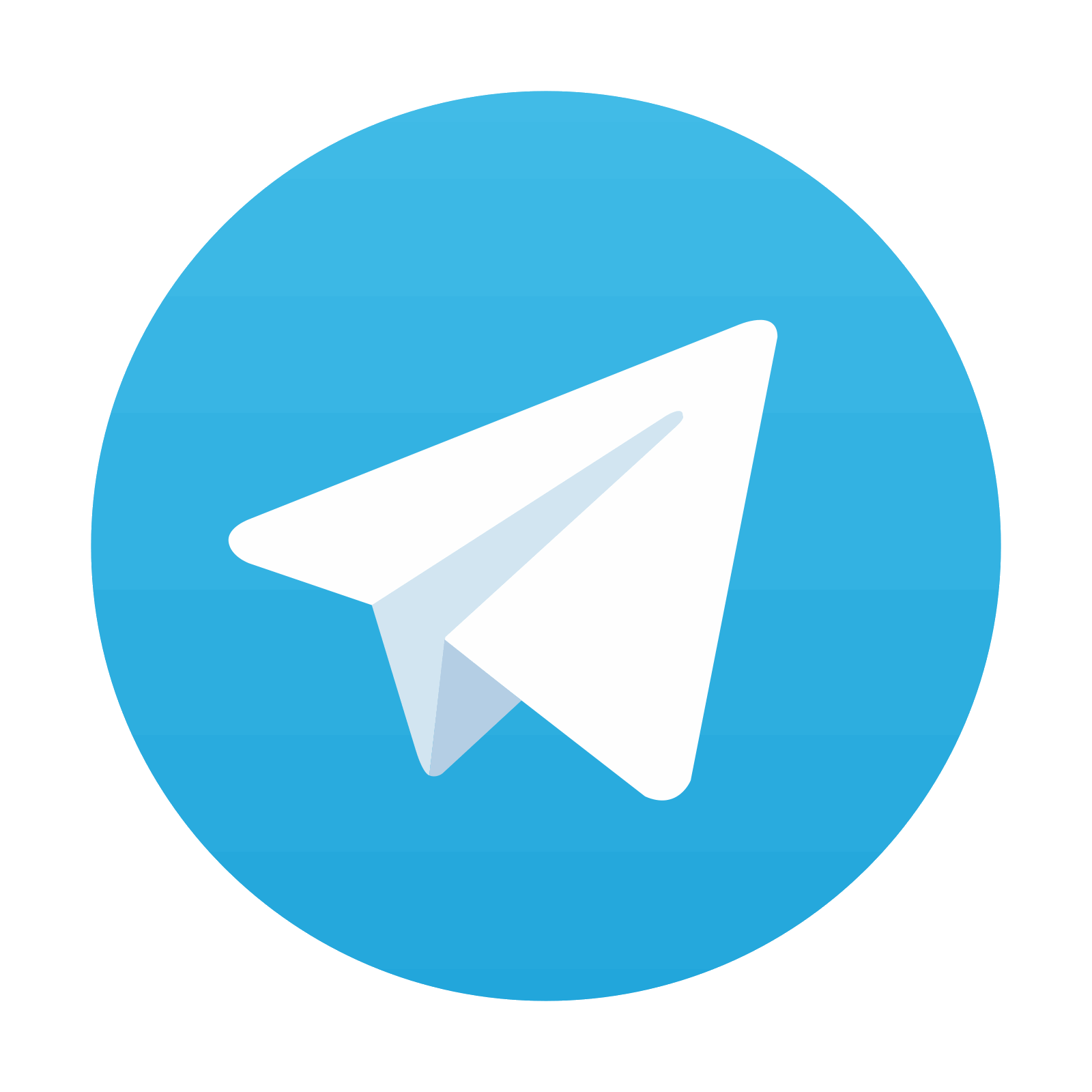
Stay updated, free articles. Join our Telegram channel
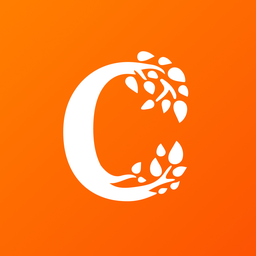
Full access? Get Clinical Tree
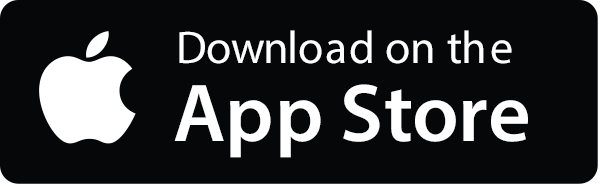
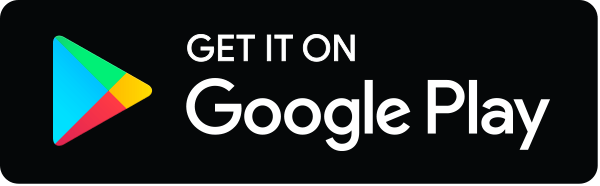