7 Commissioning, Qualification, and Validation
Christina Meyer Dell Cioppia
CONTENTS
Project and Engineering Change Control
Project Life Cycle Methodology and CQV
Project and Engineering Deliverables
Conceptual Equipment Arrangements or Layouts
Material, Equipment and Personnel Flow Diagrams
Maintenance of an Integrated Schedule
Super Skid and Skid Module Philosophy
Critical Quality Attributes and Process Parameters
Concept Phase cGMP Design Review
Piping and Instrumentation Diagrams
System Identification and Development of a Systems List
System-Level Impact Assessment
Vendor Document Requirement Matrices
Engineering Documentation Requirements
Construction Documentation Requirements
Preliminary Phase cGMP Review and Design Qualification
Finalize Templates and Review or Approval Process
Project Commissioning and Qualification Master Plan
Computer System Validation Master Plans
Laboratory and Methods Validation Master Plan
Process and Cleaning Validation Master Plan
Extractable and Leachable Testing Master Plan
Shipping Validation Master Plan
Construction Quality Assurance Plans
Commissioning and Qualification Approaches
Detailed Design Review or Design Qualification
Development of Commissioning Documents
Development and Approval of Qualification Protocols
Performance Monitoring and Earned Value
INTRODUCTION
Within the past 30 years, the pharmaceutical industry’s perception of and attitude towards validation has changed profoundly. Validation was historically viewed as a hindrance to progress and facility operation. It resulted in massive paperwork, project cost overruns, schedule delays, and operational burdens. Thirty years ago, activities related to validation generally were not even considered until well into the construction phase of a capital project. Times have changed, and now it would be unusual for a project to be initiated without considering the ultimate requirement to design, build, and hand over a validated facility. While facility designs for a validated facility are profoundly altered by the requirements of validation, project teams now recognize that most of the design elements are subject to intense scrutiny, justification, documentation, and verification. It is now standard practice to use the life cycle approach for the design, verification, validation, and operation of a facility since these are recognized as interdependent parts of a whole. The early phases of the life cycle set the stage for successful handover and operation in the future.
The word validatability has been coined to express those elements of the design that are subject to validation. Designs must be more than merely functional; they must be capable of meeting the preestablished requirements that regulatory agencies and industry standards have set for the validation of various systems. Project teams recognize that facility design and ultimate validation are intertwined. A well-structured team fosters communication and cooperation between the design, procurement, construction, quality, and validation teams.
EXECUTIVE SUMMARY
This chapter gives a brief history of what is broadly referred to as validation, but often means commissioning and qualification. This also includes an overview of the expectations of regulatory agencies and industry practices to deliver a validated facility and an overview of the evolution of validation from the 1970s to today. The concentration is on current Good Manufacturing Practice (cGMP) issues related to commissioning, qualification, and validation (CQV) during the design phase of a facility. This includes an overview of the role of the concepts quality by design (QbD) and good engineering practice (GEP) in the design process. This chapter focuses on the specific CQV activities associated with the three main phases of facility design: conceptual design, preliminary design, and detailed design.
HISTORY OF VALIDATION
Many legislative acts and regulations have been born out of tragic events that have killed or injured thousands of patients. Examples of such events are discussed below.
In 1937, sulfanilamide was used widely in tablet and powder form to treat streptococcal infections. There was a demand for the drug in liquid form, so it was dissolved in diethylene glycol and sold as Elixir Sulfanilamide, but it killed 107 people, many of whom were children. This dramatized the need to establish drug safety before marketing and was the impetus behind the passage of the Food, Drug, and Cosmetic (FDC) Act of 1938. The act required that new drugs show safety before being sold [1].
In 1941, nearly 300 deaths and injuries resulted from the use of sulfathiazole tablets, an antibiotic tainted with the sedative phenobarbital. In response, the Food and Drug Administration (FDA) drastically changed manufacturing and quality control requirements, which led to the development of cGMPs.
In 1952, nearly 180 cases of often deadly blood diseases were caused by the antibiotic chloramphenicol. Two years later, the FDA engaged the American Society of Hospital Pharmacists and the American Association of Medical Record Librarians, and later the American Medical Association, in a voluntary program of drug reaction reporting [1].
In 1962, thalidomide was sold as a treatment for anxiety and gastritis in Europe, and later to treat morning sickness in pregnant women. The drug was advertised by its manufacturer as being completely safe for everyone, including mother and child “even during pregnancy.” By 1960, thalidomide was marketed in 46 countries (but not in the United States), with sales almost matching those of aspirin [2]. Unfortunately, more than 5,000 infants in Europe and more than 10,000 infants worldwide were born with phocomelia, a malformation of the limbs. Media reports on how Frances Kelsey, MD, an FDA medical officer, helped prevent approval and marketing of thalidomide in the United States stirred up great support for the FDA and stronger drug laws. This led to the passage of the Kefauver–Harris Amendments, which required drug makers to prove that their drug worked and was safe before the FDA could approve it for sale [1].
In 1982, after several deaths from cyanide-laced acetaminophen (Tylenol) capsules, the U.S. Congress passed the Federal Anti-Tampering Act in 1983, making it a crime to tamper with packaged consumer products [1].
To understand the history of and basis for validation, it is necessary to understand the history of drug products in the United States. In the early 1800s, the United States depended largely on Europe for pharmaceuticals, which made the United States a dumping ground for foreign adulterated and even banned drugs, with often tragic results. This concern led to the publication of the first United States Pharmacopeia (USP) in the 1820s. Yet, adulterated drugs continued to make their way into the U.S. market. Public outcry grew during the Mexican–American War of 1846, when, right or wrong, adulterated drugs were blamed for high-mortality rates among U.S. soldiers. This led to the signing of the Drug Importation Act of 1848, which prohibited the importation of adulterated drugs into the United States. The act was supposed to be enforced by government-appointed inspectors stationed at key points of entry into the United States. Sadly, the law was an abject failure. In addition to the fact that inspectors were not always the best qualified for their duties, the act did not address the growing problem of adulterated domestic medicines [2].
The FDA began in 1862, when President Lincoln appointed a single chemist, Charles M. Wetherill, to serve in the Department of Agriculture. This marked the beginning of the Bureau of Chemistry, the predecessor of the FDA. The FDA’s true roots as a consumer protection agency began in 1906 with the passage of the Pure Food and Drugs Act. The act prohibited interstate commerce in misbranded and adulterated food, drink, or drugs. The Meat Inspection Act was passed the same day after shocking disclosures of unsanitary conditions in meat packing plants [3]. Today, the FDA oversees most food products (other than meat and poultry), human and animal drugs, biologically derived therapeutic agents, medical devices, cosmetics, and animal feeds. In 2013, the FDA employed more than 14,000 full-time employees [4] with an operating budget of $4.5 billion [5].
Clearly, the FDA is involved in much more than inspecting drug manufacturers and writing 483 citations, a form issued by the FDA notifying a company of objectionable conditions, however, keeping the U.S. drug supply unadulterated is a major part of the FDA’s efforts, of which validation is a key activity. Validation in the pharmaceutical industry appears to have its origins in the United States during the early 1970s. The term process validation (PV) was introduced to the pharmaceutical industry by Ted Byers and Bud Loftus of the FDA. The FDA’s objective was to enhance the quality of sterile drugs produced in the United States in response to well-publicized sterility issues with parenterals. Since validation seemed to have been an outgrowth of a major regulatory crisis, firms that did not make parenteral products were clearly skeptical of what was perceived to be an FDA overreaction to a problem unique to sterile product manufacturers. Despite these misgivings, FDA pressure was such that validation activities for sterilization processes were underway at virtually all U.S. parenteral manufacturers by the middle of the decade. The definition of validation in the 1970s provided little clear guidance as to its real intent, nor could anyone have foreseen in that definition the substantial impact validation was to have on the industry eventually.
“Validation is the attaining and documentation of sufficient evidence to give reasonable assurance, given the state of science and the art of drug manufacturing, that the process under consideration does, and/or will do, what it purports to do” (Ted Byers, June 1980).
Within this context, the industry began its first validation efforts. The goals of industry during this early period were in reaction to the lack of clarity on what validation really was and what it was required to be in a compliant state. This led to doing everything conceivable (often meaning mountains of paperwork) to “satisfy the FDA” out of a fear of the consequences of noncompliance. The solution was to keep the FDA happy and keep the facilities operating. The initial area of activity within the industry was almost totally directed toward sterilization and aseptic processing.
As firms focused on sterilization validation programs, the FDA continued to make presentations in support of validation and an industry perspective began to evolve. It was clear that the FDA intended to emphasize validation and impose it beyond just sterilization. Validation had become a part of cGMP expectations throughout the parenteral industry. Soon, the FDA recognized the merits of validation for all types of processes.
By the end of the 1970s, validation was largely a regulatory exercise that remained isolated from the rest of the firm and was certainly not an area of high concern during the design phase of a project. In 1987, the FDA published its “Guideline on General Principles of Process Validation” [6]. While there was initial opposition to the guideline’s tone, there was general consensus that validation was now a way of life for the pharmaceutical industry. Within the guideline, the FDA provided the following definition that clarified expectations:
“Process validation is a documented program which provides a high degree of assurance that a specific process will consistently produce a product meeting its predetermined specifications and quality attributes” [6].
The 1987 guidance, which was revised in 2011, specifically included active pharmaceutical ingredients: “This guidance outlines the general principles and approaches that the FDA considers to be appropriate elements of process validation for the manufacture of human and animal drug and biological products, including active pharmaceutical ingredients (API or drug substance), collectively referred to in this guidance as drugs or products. This guidance incorporates principles and approaches that all manufacturers can use in validating a manufacturing process” [6].
It is important to discuss the terms qualification and validation. While these terms are often used interchangeably, their meaning is quite different, often leading to confusion. Early on, validation became synonymous with the activities focused around protocols, data acquisition, and reports. Validation had myriad interpretations, depending on the audience. However, in the simplest terms, equipment and systems are commissioned and qualified, but processes are validated. In the context of a design guide, this chapter focuses mainly on commissioning and qualification.
Commissioning includes predelivery inspection (PDI), factory acceptance testing (FAT), site acceptance testing (SAT), and field commissioning. Qualification includes design, installation, operational, and performance qualification (PQ). These may be combined with commissioning or verification, but the focus is on equipment and systems; these activities are engineering activities. PQ is the final step before validation. Commissioning and qualification confirm and document that systems are “fit for purpose.” Following these activities, processes can be validated.
Qualification was not a significant part of many programs when validation first became a required activity in the late 1970s. The focus of regulatory guidance was on PV, which was often referred to as process qualification. Aspects of equipment and system installation and functionality were only minimally addressed. It was recognized that to ensure the reliability and consistency of validated processes, the equipment must function in a reliable manner. Measurement and confirmation of system operation could serve as a predictor of its ability to provide acceptable results in a subsequent PV study. With this, the qualification of equipment and systems as a precursor to validation became a standard feature of a sound validation program.
Validation generally refers to PV and also includes other product/process-related activities, such as methods validation, cleaning validation, mixing studies, shipping validation, dirty hold-time studies, and clean hold-time studies. These activities are critical to compliance and arguably more important than qualification: if a process cannot be validated, it is not a viable process; however, if a system cannot be qualified, the process in it cannot be validated.
In the early 1990s, there was a lack of understanding on the part of industry as to what validation really entailed and how this could add value. At the same time, the fear of 483 citations resulted in an overkill approach to qualification and validation. While the cost of qualifying and validating a facility was viewed as excessive, this was far eclipsed by the cost of a bad inspection and not being first to market.
The evolution of CQV has been driven by a number of factors, including greater industry involvement in the development of industry standards and regulatory guidelines, a greater focus on process understanding in developing and executing validation programs, and a realization that validation is not an endpoint in the project life cycle. Validation is a continuum of activities that, if properly executed, will greatly ensure product quality and patient safety, streamline regulatory inspections, and offer the opportunity for process improvements.
QUALITY BY DESIGN
In 2002, the FDA announced the pharmaceutical cGMPs for the twenty-first century. The initiative was an attempt to integrate quality systems and risk management approaches into existing programs with the goal of encouraging industry to adopt modern and innovative manufacturing technologies. This was spurred by a number of factors and goals, including (1) the need to harmonize cGMP with other non-U.S. pharmaceutical regulatory systems and with the FDA’s own medical device quality system regulations; (2) the need to make the development of innovative medical products more efficient, so that safe and effective therapies could reach patients sooner; (3) the need to stress the importance for manufacturers to use robust quality systems and appropriate process knowledge to aid in implementing process improvements; (4) the hope that effective quality systems would lower the risk of manufacturing issues, better control product quality and reproducibility, and result in shorter and fewer FDA inspections; and (5) the provision of the necessary framework for implementing Quality by Design (QbD), continual improvement, and risk management in the drug manufacturing process [7]. The overarching philosophy articulated in the FDA’s quality systems approach is, “Quality should be built into the product, and testing alone cannot be relied on to ensure product quality” [7].
QbD means designing and developing a product and associated manufacturing processes to ensure that the product consistently attains a predefined quality at the end of the manufacturing process. An essential element of the life cycle approach to CQV is the concept of QbD, which has become a catch phrase that most organizations throw around freely; QbD needs to be implemented during the product development phase through a thorough understanding of the product. QbD provides for a proactive approach to pharmaceutical development through process knowledge. At the project level, this is accomplished by understanding critical quality attributes (CQAs) of the product. CQAs are the physical, chemical, biological, or microbiological properties or characteristics that should be within an appropriate limit, range, or distribution to ensure the desired product quality. CQAs are generally associated with the drug substance, excipients, intermediates (in-process materials), and drug product [8]. Following identification of the CQAs, associated critical process parameters (CPPs), which control CQAs, are identified. There should be CPPs associated with each CQA. The design effort and subsequent project phases, including construction, commissioning, qualification, and validation, can then focus on delivering a process and facility that consistently deliver products that achieve these CQAs.
The implementation of QbD is not something that can take place as an afterthought well into design. QbD concepts include a life cycle approach, which requires that CQV be considered early and throughout the project. Input often is not provided early due to budgetary constraints, lack of resources, or the belief that the early design phases are purely engineering functions that will be impeded by CQV and quality input. This often leads to increased project costs due to rework and schedule slippage.
The project team has a shared goal: everyone shares the desire to bring safe, effective, and often lifesaving products to market; to meet project budgets and schedules; and to deliver a facility that surpasses operational and quality needs, expectations, and requirements. The continuously conflicting constraints of schedule, budget, and quality exist on every project. There is no perfect design that incorporates all operational, schedule, budgetary, and regulatory requirements into an easily validated design. These areas generally become points of contention between different project disciplines. Finding a successful balance between them is what defines a successful project.
GOOD ENGINEERING PRACTICE
GEP is key to successful implementation of QbD on any project. ASTM E2500 defines GEP as “those established engineering methods and standards that are applied throughout the life cycle to deliver appropriate and effective solutions” [9]. Examples of GEP include project and design management, the use of registered professional engineers, document control, project controls, the use of preapproved engineering specifications and standards, structured and documented design reviews, and change control. For areas and systems that are critical to product quality, GEP is supplemented by enhanced documentation, inspection, and testing activities, including qualification and validation. GEP must be applied throughout the project life cycle to deliver cost-effective facilities that meet user quality, safety, environmental, and regulatory requirements.
PROJECT AND ENGINEERING CHANGE CONTROL
Project change control (PCC) refers to the planned and documented method for the review, evaluation, approval, and communication of project changes before handover of the project to the end user. PCC is an essential element of GEP and critical to implementing QbD on any project. The purpose of PCC is to ensure that proposed changes are properly evaluated to control effects on schedule, budget, safety, system performance, user requirements, product quality, and compliance with applicable regulations. PCC is an engineering project function. Typically, the project manager is responsible for implementing PCC to ensure that (1) controls are in place to prevent changes from being made without notification of and approval by appropriate members of the project team, (2) changes are properly documented, (3) changes are properly reviewed and approved by appropriate team members, and (4) changes are communicated to appropriate members of the project team. The project team may include engineering, construction, commissioning, qualification, validation, quality, regulatory affairs, operations, maintenance, automation, and environmental health and safety (EHS), as well as system owners. Changes need to be properly closed out, with all impacts addressed, including changes to documents affected by the change.
PCC is critical to GEP; however, it can limit the progression of the project during the early project phases. During the conceptual and preliminary design phases, design of the facility is very fluid, and PCC during these early phases is not recommended. PCC is typically implemented at the completion of the preliminary design phase with issuance of “issued-for-design” (revision 1.0) documents.
PCC should not be confused with site change control or quality assurance change control. Site change control refers to the planned and documented method for review, approval, and documentation of changes to critical drawings and documents, following turnover of the project to the site system owners.
Quality assurance change control is critical to keeping systems and processes with a product quality impact in a compliant, validated state. During facility design, construction, and commissioning, changes are handled by project engineering, according to GEPs. Quality may not be routinely involved in the PCC process, since these changes are typically linked to the technical management of the project and engineering documents. However, the PCC system should allow for quality review and input into the change when the change affects a qualified or validated system, a cGMP critical document, such as user requirement specifications (URSs), or the approach to CQV (i.e., a schedule change that dictates a family approach to media-hold studies, where three media-hold studies might be conducted on the first bioreactor, with only one study conducted for each subsequent bioreactor).
PROJECT LIFE CYCLE METHODOLOGY AND CQV
The use of a life cycle methodology requires that CQV and quality considerations be raised early in the project. This proactive approach is critical to the handover of a compliant facility. It is also critical that appropriate resources, which are often in short supply, focus on areas that ultimately have an impact on product quality and patient safety. Using quality personnel to approve equipment specifications, building footprints, process calculations, and other engineering deliverables is not a wise use of their time and expertise; it is also often detrimental to schedule and budget. However, focusing these resources on high-level, product-impacting documents, including master plans, USRs, and cGMP design reviews, is certainly critical to the success of the project.
The following sections detail typical deliverables throughout the project life cycle that impact quality; these are not intended to be a comprehensive list of all deliverables through each project phase. Project phases are described in this chapter as the concept, preliminary, detailed design, execution, validation, and closeout phases. Other naming conventions are often used, such as the feasibility phase, project definition phase, basic engineering phase, or basis of design phase. Many companies have defined the specific phases and required deliverables in structured guidelines or standards. Depending on the project scope, size, schedule, and deliverables, these phases may be combined, and deliverables may fall into different phases than those presented here. Projects typically progress from one phase to the next after completion of defined “stage gates.” These stage gates must be completed before a formal decision is made to authorize progression to the next project phase. Often, these stage gates include critical deliverables, which form the foundation for later project deliverables, such as system URSs. The stage-gate approach to project phasing has become an industry-accepted practice. It has been shown to be effective in producing a quality driven design, where disciplined teams are careful to check the quality of deliverables at each gate. A formal project review should be conducted at the completion of each phase to review the status of the project, the deliverables completed during the phase, and the direction of the project against the initial project charter. Projects generally proceed to the next phase with open deliverables, with the exception of critical stage-gate items that are prerequisites for the next phase. Finally, it should be noted that the intent of the following sections is not to limit the input of CQV and quality representatives during the design phase of the project; the intent is to focus often limited resources in the areas most critical to product quality, licensing, and ultimately, patient safety.
CONCEPTUAL DESIGN PHASE
This phase begins soon after the manufacturers recognize the need for a new facility or renovation, and for large projects, it generally precedes approval of the capital appropriation request. Its purpose is to provide an initial definition of the project in terms of scope, cost, and schedule by evaluating and selecting high-level business and technical options that can achieve the business need and provide the basis for the initial confirmation of financial viability. This phase therefore generally begins with broad, high-level URS, in which the goals of the facility are described quantitatively and qualitatively.
Quantitative goals are numerical measures used to define the facility in physical terms relative to size, dimension, and layout. Quantitative goals are objective and easy to define. Qualitative goals are more subjective in nature. These include goals for compliance, such as a design that meets worldwide cGMP requirements, is highly automated, or is state of the art in terms of technology. Quality and CQV input at this stage of the project are highly recommended, since this will highlight areas where regulatory concerns may influence design. This stage of the project may take longer than expected, as management teams often challenge cost or schedule objectives, necessitating the development of other options to achieve agreement on scope, estimated cost, schedule, and functionality of the finished facility.
PROJECT AND ENGINEERING DELIVERABLES
Typical deliverables from the conceptual design phase that have a CQV impact are discussed below. These are mainly project and engineering deliverables except where noted. However, a number have a quality impact, and as such, review and approval of the items by the CQV and quality representatives are highly recommended.
User Requirements Brief
The user requirements brief is typically developed by the engineering and design team to provide a comprehensive overview of the project and key drivers, including overall business and technical objectives, scope, schedule, cost, and requirements for regulatory compliance. This sets the direction for the project and should be reviewed but not approved by the CQV and quality teams.
Block Flow Diagrams
Block flow diagrams (BFDs) show major steps in the manufacturing process. Incoming and outgoing flows are shown, with process items shown in blocks (rather than as equipment). These are precursors to the process flow diagrams (PFDs).
Process Flow Diagrams
These depict the process flow, including material and energy balances, with BFD blocks replaced by equipment. The PFDs are the precursors to piping and instrumentation diagrams (P&IDs).
Conceptual Equipment Arrangements or Layouts
These indicate the location of major equipment items in the facility and are a prerequisite to material, equipment, and personnel (MEP) diagrams. At this phase, room classifications may be shown on the classification diagrams or listed in tabular form for classified spaces. While these are engineering deliverables, these should be reviewed by quality.
Material, Equipment and Personnel Flow Diagrams
These Material, Equipment and Personnel (MEP) diagrams show the flow of material, equipment, personnel, and waste through the facility. MEP diagrams should accurately depict these flows from entry into the facility through exit. These will be refined in later phases; however, broad MEP philosophies should be established at this phase, including the following: (1) Is two-way flow acceptable in certain areas? (2) What areas need to be fully segregated? (3) Is batch-to-batch full segregation required? (4) Is temporal segregation acceptable? (5) Are combined personnel and material airlocks acceptable, or are combined entry and exit airlocks acceptable? (6) Is the flow of personnel from less clean to cleaner areas, with increased gowning requirements, incorporated into the design?
Often, MEP diagrams are accompanied by a narrative describing flows throughout the facility from entry through exit. Such descriptions are very beneficial in helping the entire project team understand gowning regimes and assumptions, airlock considerations, cross-flows, and potential areas of concern with the overall design. A meeting is also helpful if a facility design meeting is going to be scheduled with the regulatory agency (see the Regulatory Review Meeting section of this chapter). It is critical to review owner design guidelines and quality directives in the review of MEP diagrams. Often, these stipulate requirements that are more stringent than agency requirements.
LEVEL II SCHEDULE
The project schedule developed at this phase establishes overall project milestones and priorities. Input from CQV should ensure that enough time is included in the schedule for all CQV activities and precursors (e.g., system prerequisites, calibration, mechanical completion, developmental runs, engineering runs, water system PQ activities, environmental monitoring, process and cleaning validation, and retest).
MAINTENANCE OF AN INTEGRATED SCHEDULE
Maintaining an integrated schedule is critical to achieving the overall project schedule and ultimately cost objectives. Separate, independent schedules are of little, if any, value. The integrated schedule should include timelines for engineering, procurement, construction, start-up, commissioning, qualification, developmental runs, turnover, validation, and regulatory filing and approval, with increasing levels of detail provided as the project progresses.
SUPER SKID AND SKID MODULE PHILOSOPHY
This consists of an evaluation of the potential to modularize certain elements of the project for the purpose of ease, speed of construction, inspection, testing, and scheduling. While this is certainly an engineering deliverable, it is a critical item for the CQV team, since it will have an impact on the system identification listing, system impact assessments, and overall inspection and testing approach.
Concept Phase Estimate
At this phase, this is generally a ±20%–30% estimate, depending on the level of deliverable completion or company practice. For CQV-related activities, a factored, not a detailed estimate, is applied. Depending on company practice, project size, scope, and type of facility, the level of engineering required for this phase may be in the range of 5%–25% and could represent 1%–5% of the total installed cost of the project.
Regulatory Strategy Document
This identifies the agencies and governments with which the facility will be licensed, their governing codes, and the related submission and inspection strategies to be followed by the project. The CQV strategy document would then be aligned with the regulatory strategy.
CQV Strategy Document
This document describes the strategy and approach that will be taken for the CQV of the new facility and for maintaining the facility in a cGMP-compliant manner. The strategy document often points to the concepts that will be used for CQV activities throughout the project; for example, “The overall strategy outlined in this document employs the concepts outlined in the ASTM E2500, ‘Standard Guide for Specification, Design, and Verification of Pharmaceutical and Biopharmaceutical Manufacturing Systems and Equipment’ and the ISPE Baseline Pharmaceutical Engineering Guide for New and Renovated Facilities, Volume 5, Commissioning and Qualification” [9, 10]. This is a high-level document that does not describe specific systems and may sit over or under the site validation master plan (SVMP). A separate CQV strategy document is not always developed. Often, the elements of the CQV strategy document are included in the site CQV master plan or project-level master plans.
Critical Quality Attributes and Process Parameters
Process understanding is a basic tenet of the life cycle approach to CQV and product quality. This is the basis for facility design. Process understanding is critical to understanding the risks that may be present in the process and mitigating these risks in the design. Key to process understanding is an understanding of CQAs and CPPs. The International Conference on Harmonisation (ICH) Q8 definitions follow: (1) The CQAs are physical, chemical, biological, or microbiological properties or characteristics that should be within an appropriate limit, range, or distribution to ensure the desired product quality. (2) The CPPs are process parameters whose variability has an impact on CQAs, and therefore should be monitored or controlled to ensure the process produces the desired quality [8]. The CQAs are identified during product development, with the process then further developed by conducting multivariate experiments to consistently produce a product meeting CQAs. To consistently deliver a product meeting CQAs, it is critical to understand the impact of material attributes and process parameters on CQAs, and then identify, control, and monitor the sources of variability in materials and the process. With this, there should be CPPs associated with every CQA. Wherever feasible, the design should allow monitoring of CQAs. This can allow for process control strategies that provide process adjustment capabilities to ensure control of all critical attributes. The CQAs and CPPs should be incorporated into URSs, which are the basis for all subsequent activities related to facility design, commissioning, qualification, validation, and handover.
SYSTEM-LEVEL URSS
Ideally, system-level URSs would be available at the completion of the conceptual phase and include CQAs and CPPs. The purpose of the URS is to describe the system intent, in terms of high-level performance requirements, including those related to cGMPs. The URS documents are used to ensure that cGMP regulatory expectations, CQAs, CPPs, and owner quality directives are incorporated into the design. It is very important to note that user requirements are not synonymous with design requirements. User requirements should focus on what is needed, without describing how the requirements will be achieved. Design requirements may be more stringent than user requirements to ensure that additional safety factors are designed into the system. User requirements should never be more stringent than design requirements.
A decision should be made at the project level as to which systems will require separate URS documents. For systems that do not have a quality impact (e.g., plant utility systems) and simpler pieces of equipment (e.g., standard refrigerators, freezers, and incubators), URSs may be incorporated in equipment specifications. All requirements indicated in the URS should be verified during design reviews and later field verified during CQV as appropriate. Non-cGMP requirements (i.e., safety, maintenance, operability, and expandability) may or may not be included in URS documents, depending on project or owner procedures and guidelines.
Engineering typically interviews user groups to determine and document user requirements on a system-by-system basis. Sources of user requirements may include the following: (1) product license requirements for an approved product; the product license stipulates critical process criteria and ranges; (2) the CQAs and CPPs and ranges defined in technology transfer documents; (3) owner guidelines and procedures; often, owner procedures stipulate more stringent requirements than regulatory bodies, so it is critical that there is parity between URS and the most stringent requirements; (4) Guidance issued by regulatory agencies, such as the FDA and European Medicines Agency (EMA); (5) the USP and International Organization for Standardization (ISO) standards and ICH guidelines; and (6) material compatibility requirements.
When documenting the user requirements, it is important to avoid overspecifying; for example, the URS might include a requirement that vessel internals are thoroughly wetted by spray balls. However, the URS should not specify the number of spray balls or rotation requirements. Overspecification leads to overly stringent requirements that are often unnecessary. This often leads to schedule delays and cost overruns. Overly detailed URS documents that go beyond performance lead to excessive documentation. Design review or design qualification needs to verify each item, which typically leads to editing and reapproving URS documents under PCC procedures. This can have a tremendous impact on cost and schedule.
Avoid underspecifying with statements that are too vague. For example, statements such as “the system must meet cGMP requirements” do not specify a particular user requirement. “Nice to have” items should also be avoided, as these are convenience items that may be desirable but are not truly cGMP critical. These items are often the focus of value engineering and often removed from the design at a later date.
It is important to include ranges. User requirements should not stipulate set values or overly stringent acceptance criteria; rather, URSs should include an acceptable range of values for critical attributes and parameters.
In the author’s opinion, URSs are among the most important deliverables of the project. The URS documents are the foundation of all subsequent design deliverables and field verification activities. They are also generally one of the key deliverables required at the stage gate to go from the concept to the preliminary phase. Unfortunately, these are often rushed because of schedule pressures, copied from previous projects, and not given the attention they deserve.
CONCEPT PHASE CGMP DESIGN REVIEW
The cGMP design review completed at this phase examines and documents applicable regulatory requirements (e.g., FDA, EMA, Canadian, and owner), as well as expectations for the overall facility. These regulatory requirements, as well as CQAs and CPPs, should be verified as being incorporated into user or system requirements and other available design documents during this initial review.
The design effort will not have progressed to allow for a review of each individual system. Rather, this review focuses on the overall facility and examines high-level arrangements and flows to ensure that a practical approach has been taken to ensure adherence to cGMP concepts, as well as agency and owner regulatory requirements and expectations. Key areas examined during this phase include (1) material receipt, quarantine, release, and reject; (2) MEP flow diagrams; (3) layout diagrams; (4) gowning regimes; (4) area classifications; (5) user requirements; (6) heating, ventilation, and air conditioning (HVAC) zoning diagrams; (7) airflow diagrams; (8) cGMP philosophies; and (9) automation philosophy.
The design review team should consist of key members of the project team, including project, engineering, automation, quality, validation, manufacturing personnel, EHS, and other subject matter experts (SMEs) as appropriate. At the completion of the cGMP design review, a report should be issued summarizing the areas examined, regulatory requirements and expectations, reference documents, decisions made, and potential risks.
PRELIMINARY DESIGN PHASE
The objective of this phase is to refine and converge technical options to identify a single project concept and develop this concept to generate issued-for-design documents. During this stage, technical documents from the concept phase are detailed, with additional deliverables developed in accordance with the deliverables list. Deliverables completed during this phase should be approved for design. Once documents are issued as revision 1.0 (or 0.0), PCC procedures should be put into place. Deliverables impacting CQV activities are described below.
PIPING AND INSTRUMENTATION DIAGRAMS
P&IDs depict all equipment, ancillary items, instrumentation and controls, piping, valves, equipment and instrument tags, fluid flows, tie-ins, and notes to give a detailed view of process, utility, and support systems. These are developed from PFDs. The P&IDs are purely engineering deliverables and need not be approved by CQV or quality. However, these drawings should be provided to the CQV team for review, since they are key reference drawings against which systems are compared during commissioning and qualification. The P&IDs should be reviewed against URS documents during design reviews, to ensure that CQV expectations are incorporated. This would include items such as sample valves, minimum distances, slopes, block and bleed valves on clean-in-place (CIP) circuits, and use point filters. Once P&IDs are approved, change management should be imposed to ensure that affected members of the design and CQV team are notified when a change is requested.
Basis of Design Documents
Basis of design (BOD) documents provide a description and the design criteria for key areas, including HVAC, process, automation, process, process utilities, plant utilities, electrical, and support areas. For example, the BOD for HVAC would describe temperature and relative humidity ranges, classifications, air-change rates, differential pressures, controls, automation, and the design basis. BOD documents with a cGMP impact (e.g., those for HVAC, process utilities, automation, and CIP) are often referenced in deliverables for GxP systems; however, these are purely engineering deliverables and should not require quality approval.
System Identification and Development of a Systems List
This is typically a CQV deliverable, with input from the project team, including system owners, engineering, and construction. This involves dividing the facility, project, or area into logical systems. A number of factors should be considered when developing the systems list, including skid or module philosophy, vendor or subcontractor scope, construction schedule, system owner, delayed installation of use point equipment, and the impact of the system on product quality.
Depending on the above, systems may be identified on a P&ID basis (i.e., each P&ID is a separate system), skid basis (i.e., all items within a skid are considered one system), vendor or subcontractor scope basis (i.e., purified water generation separate from storage and distribution), or a system impact basis (i.e., if there are very few quality-critical components within a particular system, include those components within the scope of a different quality-critical system). Systems should be numbered in accordance with a company standard nomenclature, and referenced throughout the project life cycle. To the fullest extent possible, the same system boundaries should be maintained during construction, commissioning, and qualification.
SYSTEM-LEVEL IMPACT ASSESSMENT
The process of completing an impact assessment was first formally presented in the ISPE Baseline Guide Commissioning and Qualification (2001). Although this was first presented over 15 years ago, before the launch of the FDA’s pharmaceutical cGMPs for the twenty-first-century initiative and before more formal and detailed procedures for conducting risk assessments became popular, most companies still use some form of the system-level impact assessment as presented in the original ISPE Baseline Guide. The impact assessment process is used to determine which systems should be subject to qualification practices in addition to GEP. Using this approach, systems are classified according to the definitions below.
Direct-Impact System
A system that is expected to have a direct impact on product quality is designed and commissioned in line with GEP and subject to additional qualification practices. A system is defined as direct impact if it meets any of the following criteria: (1) it comes in contact with the product (e.g., air quality); (2) it provides an excipient or produces an ingredient or solvent (e.g., water for injection [WFI]); (3) it is used in cleaning or sterilizing (e.g., clean steam); (4) it provides status preservation (e.g., nitrogen); (5) it produces data that are used to accept or reject product (e.g., electronic batch record system or CPP chart recorder); or (6) it is a process control system (e.g., programmable logic controller or distributed control system) that may affect product quality, and there is no system for independent verification of control system performance in place [10].
Indirect-Impact System
An indirect-impact system is one that is not expected to have a direct impact on product quality, but typically will support a direct-impact system. Example include plant steam and chilled water. These systems are designed and commissioned following GEP, and not subject to qualification practices.
No-Impact System
A system that will not have any impact, either directly or indirectly, on product quality is a no-impact system. These systems are designed and commissioned following GEP.
The system-level impact assessment is a CQV deliverable that should be approved by quality.
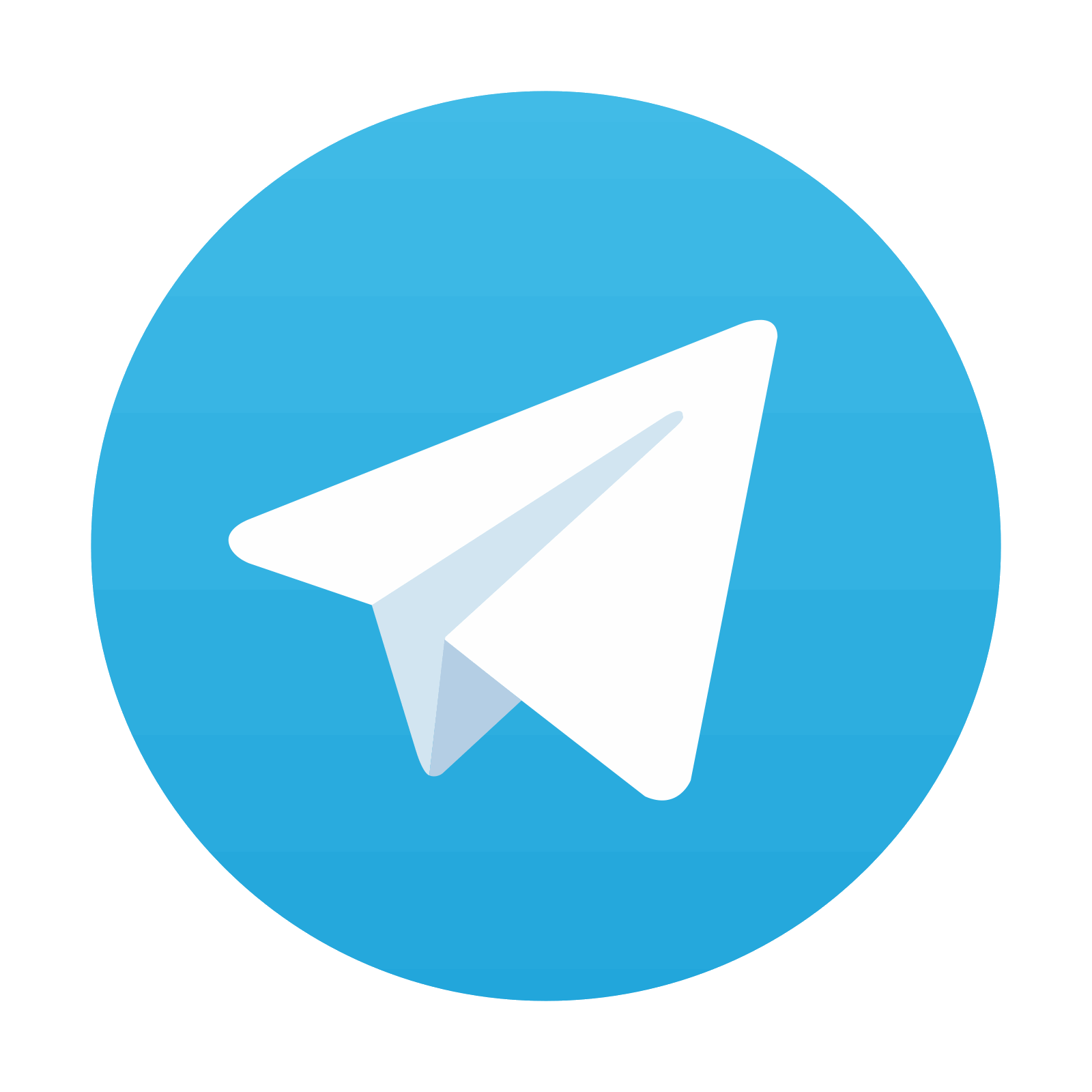
Stay updated, free articles. Join our Telegram channel
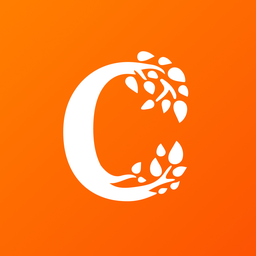
Full access? Get Clinical Tree
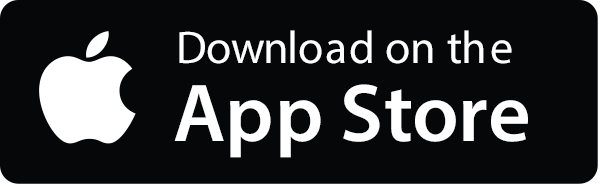
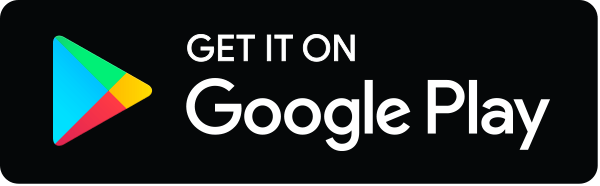