Chapter 4
Coherent Raman for Medical Diagnosis
4.1 Introduction
Traditional diagnosis of tissue pathology involves biopsy followed by ex vivo examination of the tissue preparation with light microscopy. An important step in this procedure is the application of stains, that is, a preparation of dyes that color specific tissue components. The most widely used staining protocol in histopathology is the application of two dyes called hematoxylin and eosin Y, jointly known as the H&E stain. Hematoxylin accumulates preferentially in the cellular nucleus, coloring the nuclei with a dark blue hue. Eosin binds predominantly to proteins, assigning protein-rich areas a red to pink color. As the cytoplasmic regions of cells are mostly eosinophilic, application of the H&E stain gives rise to tissue images in which cells can be clearly recognized by the red/pink coloring of their cytoplasm while the nuclei stand out as blue. Stains like H&E make it possible to characterize cellularity in the tissue and recognize tentatively deviant cell morphologies.
Staining protocols are important; without proper coloring of tissue components, histopathological analysis would be virtually impossible. Nonetheless, standard histopathology is not only a time-consuming practice but also invasive for the patient. Therefore, approaches that shorten the time duration to come to a diagnosis and minimize the invasiveness of the method are highly desirable. This has led to alternative visualization methods that can complement and possibly replace standard histopathology protocols.
An important step toward modernizing histopathological practice is to avoid the need for staining the tissue. Because staining cannot be performed on live tissue, the need for coloring the tissue with stains such as H&E stipulates that histological inspection is always coupled to biopsy. Real improvement can be expected if the visual tissue contrast can be attained without the necessity of ex vivo staining. This would not only shorten the time of the diagnostic procedure but also offer the ultimate possibility of performing the diagnosis directly in vivo.
Optical spectroscopic methods are the primary candidates to replace traditional staining. Using the spectroscopic contrast of endogenous tissue components, optical methods can provide chemical maps of the tissue in a stain-free and noninvasive matter. Several optical techniques have shown to hold promise in this regard. Fluorescence microscopy, for instance, can visualize cell structures and elastin fibers in tissues. Unfortunately, only a few native tissue components show sufficient fluorescence response that is suitable for imaging purposes, ultimately limiting the contrast that can be achieved in this fashion. Raman microscopy is another imaging modality that is sensitive to the chemical makeup of endogenous tissue compounds. Unlike fluorescence spectroscopy, Raman spectroscopy identifies structures based on their unique molecular vibrations. As virtually all molecules exhibit vibrations of this kind, Raman spectroscopy is not fundamentally limited to visualizing just a few tissue components. Because of this reason, imaging based on Raman contrast holds great promise as a general label-free visualization tool for tissue examination.
Despite the very attractive form of contrast that Raman microscopy provides, this approach has not yet found wide implementation for tissue diagnostics. The reason for this is the image acquisition rate of the Raman microscope, which is several orders of magnitude slower than what is needed for practical tissue diagnosis. Fortunately, new developments in the area of Raman imaging have addressed the issue of slow imaging rates. The most exciting development in this direction is the use of so-called coherent Raman scattering (CRS) techniques (Figure 4.1). These are imaging techniques that feature the same kind of contrast as the regular Raman microscope but induce nonlinear optical processes in the sample to boost the signal levels by multiple orders of magnitude. Consequently, CRS microscopy techniques have matured into reliable label-free imaging approaches that are very much in line with the stringent requirements for clinically significant examination of tissues (Figure 4.2).

Figure 4.1 Multichannel SRS images. Images were pseudocolored to highlight different cell compartments. (a–c) A live salivary gland cell of fruit fly Drosophila; a breast cancer cell (MCF-7) and human embryonic kidney cells (HEK-293) in culture. Of note, nucleic acids inside the cell nuclei are visualized by probing the phosphodiester stretching vibration (785 cm−1) and dioxy stretching vibration of the phosphate backbone (1090 cm−1). Contrast from other cellular compartments, including the cell bodies and lipid droplets, originates from the CH2 stretching vibrational modes.

Figure 4.2 Increasing usage of CRS as a biological imaging tool. Keywords used in PubMed Search: Raman, CARS, microscopy, and tissue.
In this chapter, the use of CRS imaging for biomedical studies is highlighted. After briefly discussing the underlying principles of CRS imaging in Section 4.2 and Section 4.3, Section 4.4 explains how the acquisition speed of CRS imaging can be combined with meaningful spectroscopic information to improve the diagnostic capabilities of the technique. In Section 4.5, several successful applications of CRS microscopy in the area of ex vivo tissue examination are discussed, which is followed by a summary of current in vivo applications in Section 4.6. The chapter concludes with a brief overview of future developments and biomedical applications of CRS imaging techniques.
4.2 Raman Contrast for Tissue Imaging
4.2.1 Origin of Chemical Contrast
Intramolecular vibrations, including molecular bond vibrations, occur at particular frequencies that lie in the 10–100 THz range. The frequencies of certain vibrations, or modes, are specific enough to use them as identifiers for the presence of chemical groups in the molecule. Hence, the mode frequencies in this range can be used to retrieve chemical analytical information about the molecules present in a sample.
Some of the mode vibrations in a molecule can be seen with optical spectroscopy. The most direct way to probe molecular vibrations is by tuning light in the 10–100 THz range into resonance with the frequency of a mode in the molecule. This leads to absorption of the light at particular frequencies, and the corresponding absorption spectrum can thus be used to retrieve chemical information about the molecules. This is the basis of infrared (IR) spectroscopy.
There are also modes that can be probed with the Raman effect. Unlike the IR absorption process, the Raman excitation is not based on the direct absorption of a photon. Instead, the Raman effect is an off-resonant excitation. This implies that the light–matter interaction in Raman spectroscopy is a lot weaker than in IR absorption spectroscopy. It also implies that modes of different symmetry can be seen. The main advantage of the off-resonant excitation, however, is that the incident light does not have to be in the IR range. Visible light can be used, which means that regular microscope optics, optimized in the visible range of the spectrum, can be used for performing Raman measurements.
What kind of molecular modes can be seen with the Raman effect? Unfortunately, not all modes can be probed with Raman spectroscopy. Only vibrational modes of certain geometries can be seen. The geometry of the mode has to be such that the vibrational motion changes the polarizability of the molecule. In practice, this condition holds for chemical group vibrations that are highly symmetric. In Table 4.1, a list of common modes of biologically relevant molecules is given. It is clear from the list that a great deal of information about molecules can be attained on the basis of the presence of certain chemical groups in the compound. The beauty of Raman spectroscopy is that this chemical information can be acquired without the use of extrinsic labels. Raman microscopy is, therefore, a genuine label-free method for generating chemical maps of biological samples.
Table 4.1 List of chemical bond assignments.
Raman shift (cm−1) | Assignments | References |
479 | C–C–C deformation | [2] |
510–540 | S–S, disulfide conformation | [3] |
722 | –N+(CH3)3 symmetric stretch | [3] |
783–790 | Symmetric phosphodiester stretch | [1, 4] |
Ring-breathing modes of pyrimidine | ||
O–P–O | ||
865 | O–C–C–N symmetric stretch | [2] |
873–883 | C–O–H | [3] |
961 | P–O4 symmetric stretch | [4] |
973 | –N+(CH3)3 asymmetric stretch | [3] |
1006 | –C=C– phenylalanine aromatic ring | [1] |
1050–1160 | –C–C–, =C–H | [1, 5, 6] |
1082–1120 | C–O–H deformation | [2] |
1200–1350 | Amide III, –N–H bend/C–N stretch | [2, 3] |
1300 | CH2 twist | [2] |
1440–1473 | CH2, bending or scissors | [2, 3] |
1480–1575 | Amide II, –N–H bend/C–N stretch | [3] |
1518–1537 | –C=C– conjugated | [2, 5] |
1630–1690 | Amide I | [1, 3] |
2570 | –S–H | [3] |
2845–2852 | CH2 symmetric stretch | [1, 3, 7, 8] |
2853–2940 | CH2 asymmetric stretch | [4, 7, 8] |
2870–2935 | CH3 stretch | [4, 7, 8] |
2960–2970 | CH3 asymmetric stretch | [4, 7, 8] |
3008–3023 | =C–H stretch | [2, 4] |
3300–3350 | O–H stretch | [4] |
The chemical contrast is, however, not solely determined by the mere presence of certain chemical groups. The Raman signal also depends on the Raman cross section of a particular mode and on the density of the respective chemical groups. The density of chemical moieties, in particular, is a governing factor. The more Raman-active chemical groups of a particular kind there are in the probing volume, the stronger the signal. Chemical groups that are present at a high density in biological molecules in tissues, such as the methine (CH), methylene (CH2), and methyl (CH3) groups, will thus produce strong Raman signals. Other important chemical moieties, such as S–H and S–S bonds in proteins, are present at much lower densities and, consequently, yield much lower Raman signals.
Another limiting factor is that many Raman spectroscopic signatures partially overlap in the vibrational spectrum. For example, in the region between vibrational energies of 800 and 1800 cm−1, the so-called fingerprint region, many relevant bond vibrations can be found. Yet, several of the vibrational signatures partially overlap, producing broad and featureless band profiles, which complicates the chemical analysis. Another example is found in the C–H stretching region, from 2800 to 3100 cm−1. Although the Raman signal is generally high in this region, the different types of C–H vibrations strongly overlap, which makes it difficult to extract quantitative information about the density of methine, methylene, and methyl groups.
Despite these complications, the chemical contrast provided by Raman spectroscopy and microscopy offers invaluable label-free information about the distribution of key molecular classes in tissues. In the next section, we highlight several examples of biologically relevant constituents in tissue that can be identified with Raman-based contrast.
4.2.2 Endogenous Targets for Raman-Based Imaging in Tissues
Many biomolecular compounds are organic molecules. This implies that the list of different types of chemical bond vibrations is limited to those of the chemical groups in organic molecules. Nonetheless, subtle differences in the Raman response of different classes of molecules help discriminate between important tissue components (Table 4.2). These differences arise because different molecular classes have (i) different densities of molecular groups and (ii) differences in their chemical environment giving rise to shifted vibrational frequencies and distinct line shapes.
Table 4.2 Tissue components.
Typical band used | Prominent vibrational | References | |
in imaging | modes | ||
Nucleic acids (DNA, RNA) | ∼785 | O–P–O | [1] |
∼2935 | C–H3 stretch | ||
Water | ∼3300 | O–H stretch | [9] |
Protein (collagen, elastin, and globular protein) | ∼1655 | Amide I | [10–12] |
∼2850 | CH2 symmetric stretch | ||
∼2940 | CH2 asymmetric stretch, CH3 stretch | ||
Carbohydrates (glucose and polysugars) | 1082–1127 | C–O–H deformation | [2, 4, 13] |
∼2870 | CH2, CH3 stretch | ||
Carotenoids (lycopene, β-carotene) | ∼1160 | =C–H stretch | [4, 6, 14] |
1510–1525 | –C=C– conjugated | ||
Lipids (cholesterol, fatty acids, triglycerides, phospholipid, and myelin) | ∼1440 | CH2 bend | [12, 15–17] |
∼2850 | CH2 stretch (aliphatic lipid chain) | ||
∼2970 | CH3 stretch (cholesterol and cholesterol ester) | ||
∼3020 | =C–H stretch (unsaturated fatty acid) |
Consider, for example, the C–H stretching vibrational range. Because of the strong Raman response, this range contains one of the most important sets of vibrational modes targeted in CRS imaging applications. Despite the fact that many biomolecular tissue components exhibit C–H stretching mode vibrations, the modes in this range can still be used to selectively probe relevant classes of molecules [18]. Figure 4.3 shows the Raman spectra of several biomolecular targets in the C–H stretching range. Although the bands are broad and overlap significantly, distinct features can be recognized for each of the classes. Importantly, areas in the sample with high protein concentrations can be discriminated from those with high lipid concentrations when comparing the relative spectral density of the symmetric methylene mode at ∼2850 cm−1 (prominent in lipids) with the spectral density around 2940 cm−1 (prominent in proteins). Even among the lipid class, which is rich in methylene groups, different subclasses can be identified in this crowded spectral region. For instance, saturated and unsaturated lipids can be discriminated from each other by means of the distinct contribution of the vinyl mode (3010–3050 cm−1), which is present only in unsaturated lipids. Also, high concentrations of cholesterol can be revealed on the basis of the presence of a distinct set of modes. These examples illustrate that many important components can be identified in the sample, allowing meaningful chemical mapping of tissue specimens (Figure 4.4).

Figure 4.3 Characteristic Raman signatures of biological compounds. Shown here are spectra in the carbon–hydrogen stretching regions (2800–3050 cm−1). Even in this relatively small spectral window, striking differences between protein-rich and lipophilic compounds, as well as between pure cholesterol and aliphatic fatty acids, can be seen. Highlighted peaks at ∼2850 and 2945 cm−1 (CH2 and CH3 mode) are commonly used in a high-speed coherent Raman imaging experiment to visualize the presence of lipid and protein density, respectively.

Figure 4.4 Label-free images of iliac arteries harvested from atherosclerotic Ossabaw pigs. Different stages of atherosclerosis were detected using label-free CARS microscopy. Images were acquired by probing the CH2 stretch mode. Hence, the bright spots correspond to lipid-rich regions within the arteries. (a–e) Type I, II, III, IV, and V atherosclerotic lesions. L, lumen; TI, thickened intima; LP, lipid pools; and LC, lipid core.
4.3 Coherent Raman Scattering
4.3.1 Basic Principle
The most direct way to measure the Raman response of tissue samples is through measuring the spontaneous Raman spectrum. The method of measuring a spontaneous Raman spectrum has not fundamentally changed since Sir Raman measured his first spectrum in 1928. It involves a monochromatic light source for illumination of the sample and a dispersive element for spectrally recording the scattered light. The measured Raman light, which appears spectrally shifted from the incident light, scales linearly with the intensity of the light source: stronger Raman signals are attained when turning up the illumination intensity. Because of this linear dependence, the Raman effect is classified as a linear optical technique.
CRS, on the other hand, is a nonlinear version of spontaneous Raman scattering: the signal strength depends nonlinearly on the illumination intensity. In many ways, CRS is a more complicated technique than spontaneous Raman scattering. Nonetheless, it offers a very important advantage: under certain conditions, much stronger signals can be obtained, which, in turn, enables a significant improvement of the imaging speed.
What is the underlying principle of the stronger signals? The answer is found in the coherent motion of the molecular group vibrations. In spontaneous Raman scattering, the molecular modes are excited by the light source. During this excitation, a photon is emitted, which constitutes the Raman signal on the detector. Each molecular mode will assume a vibrational oscillation with a phase that is independent of the vibrational phase of neighboring molecules. In other words, the molecules do not oscillate in a synchronized manner. This also implies that the emitted photons are not synchronized; they have a phase independent of all the other emitted photons. Because of this lack of synchronicity, the resulting emission is said to be incoherent. The situation is different in CRS. Now the molecules are actively driven with two laser beams that make them oscillate in step. The synchronized oscillation of the molecules produces a coherent optical signal. The difference between the spontaneous Raman signal and the CRS signal is like the emission from a light bulb versus the light from a laser source: in the first case, the light is incoherent and scattered in all directions, and in the second case, the light is coherent and highly directional. The coherent light is particularly strong in the so-called phase-matched direction. When detected in this direction, the CRS signal can be many orders of magnitude stronger than the corresponding incoherent Raman signal (Figure 4.5).

Figure 4.5 (a,b) Signal generation in spontaneous Raman scattering and in CRS. During a spontaneous Raman process, emitted Raman photons from the molecules have independent phases, thereby resulting in an incoherent and weak signal. In CRS, the molecules oscillate in unison, where the emitted Raman photons interfere constructively, producing much enhanced signals.
How much stronger is the CRS signal? This depends on the conditions. Consider a lipid droplet with a diameter of 1 μm. In the CRS microscope, the signal of the methylene stretching mode from such a droplet is about four orders of magnitude higher than what one would measure in the spontaneous Raman microscope using the same average illumination intensity. This translates into a pixel dwell time of 1 μs for CRS versus 10 ms for spontaneous Raman. Clearly, this is a very attractive speed advantage for CRS imaging. However, this speed advantage currently holds only for single (vibrational) mode measurements. When spectral information over a wider range is required, the CRS pixel dwell time progressively increases. Hence, the use of CRS microscopy is most relevant when the spatial distribution of a given vibrational mode holds important clues toward tissue composition. For instance, when the distribution of lipids in the specimen is the prime target of the imaging study, then the strong signal from the methylene stretching mode can be used as a vibrational marker. Under such conditions, full advantage can be taken of the high image acquisition speed of the CRS microscope. Clearly, the balance between acquisition speed and spectral information is an important parameter in CRS imaging. In Section 4.4, we will discuss this point in greater detail.
4.3.2 Coherent Anti-Stokes Raman Scattering (CARS) versus Stimulated Raman Scattering (SRS)
Several techniques fall into the category of CRS imaging. The most important members of the CRS family are coherent anti-stokes Raman scattering (CARS) and stimulated Raman scattering (SRS) microscopy [20–22]. Other members include optical Kerr effect (OKE) [9] and Raman-induced Kerr effect (RIKE) microscopy [23], but these latter technologies have not yet matured into an imaging technique for rapid visualization of tissue samples. CARS and SRS, on the other hand, have been shown to be well suited for chemical imaging of tissues.
The vibrational contrast in both CARS and SRS microscopy originates from the Raman response of the tissue components. Hence, in terms of chemical information, CARS and SRS probe identical parameters: the Raman active modes of the molecules. Nonetheless, there are important differences between the two techniques. Some of these differences are fundamental in nature, while others are primarily practical. A comparison of the techniques is given in Table 4.3.
Table 4.3 Comparison between the imaging properties of CARS and SRS.
Imaging property | CARS | SRS | Comments |
Lateral spatial resolution | ∼0.3 μm | ∼0.3 μm | Comparable to confocal microscopy |
Axial spatial resolution | ∼1.0 μm | ∼1.0 μm | Comparable to confocal microscopy |
Pixel dwell time | <1 μs | <1 μs | Video-rate compatible, narrowband CRS only |
Epi-detection sensitivity | High | Moderate | Important for live tissue applications |
Concentration dependence | Nonlinear | Linear | SRS is more quantitative |
Background contribution | Strong | Weak | SRS offers the sharpest chemical contrast |
Background origin | Electronic Kerr response | Pump–probe, photothermal | Background in CARS offers morphology contrast |
Two-photon fluorescence background | Yes | No | Fluorophores can be seen through pump–probe in SRS |
Deconvolution for image restoration | No | Yes | More relevant for cellular imaging than for tissue imaging |
On a fundamental level, SRS microscopy measures, in principle, only the Raman response of the molecules. The SRS image contrast is, therefore, similar to the contrast one would expect from spontaneous Raman images. CARS microscopy probes the same Raman response, but, in addition, it detects a signal component that originates from the electronic properties of molecules rather than their vibrational properties. This latter contribution, often referred to as the nonresonant background in CARS, is present regardless of the vibrational contribution of the signal. Importantly, the vibrational and nonresonant background signals do not appear as a simple addition in CARS. Instead, the two signal contributions are mixed in a nonlinear manner, which makes it challenging to reliably disentangle the two. The presence of the nonresonant background is the primary reason why SRS is sometimes favored in certain applications over CARS microscopy [22].
However, for some tissue imaging applications, the presence of the nonresonant background can be very meaningful. In particular, although chemically unspecific, it provides a high contrast map of the tissue density. For instance, the contrast is reminiscent of the density-related (non-chemical) contrast in optical coherence tomography (OCT) and in confocal reflectance microscopy, both of which have proven to be very useful for mapping tissue architecture and morphology. The nonresonant CARS signal provides comparable information albeit with a much higher imaging resolution than what can be obtained with OCT imaging and with much finer contrast than what can be seen with confocal reflectance microscopy.
On a practical level, both SRS and CARS imaging feature high 3D imaging capabilities, along with fast image acquisition rates (Figure 4.6). One advantage of CARS over SRS is that the signal levels in CARS microscopy detected in the backward (epi-)direction are generally stronger. This CARS feature may be useful when imaging live tissue samples, in which case epi-detection is the only feasible detection strategy. However, it should be noted that this issue is a practical rather than a fundamental limitation, and subsequent technological developments may improve the epi-detection in SRS microscopy [12].

Figure 4.6 Three-dimensional SRS reconstruction of live chordate. (a) Forty-five SRS images were acquired in vivo at 2950 cm−1 to generate a 3D map of living amphioxus’ head. Length of the 3D image along the longitudinal axis is approximately 738 μm. (b) Protein-rich structures, including myofilaments, collagen, and muscle-like cells, were resolved with submicrometer spatial resolution. No prior tissue sectioning was performed.
Which CRS technique is more useful for a given tissue imaging application? That depends on the type of application. However, it should be noted that a CRS microscope automatically generates both SRS and CARS. It is common practice to collect both signals simultaneously. In this way, no a priori choice has to be made between CARS and SRS contrast. Both channels contain useful information, and it is usually the combination of CARS and SRS that offers the microscopist access to various important aspects of the tissue: high-resolution morphological information with CARS and sharp chemical contrast with SRS (Figure 4.7).

Figure 4.7 CARS and SRS contrasts of live C. elegans. (a,b) Both CARS and SRS channel reveal the presence of lipids when probed at the CH2 stretch mode of 2845 cm−1. (c,d) The presence of nonresonant background at off-resonance (2796 cm−1) in the CARS channel provides morphological contrast of the tissue architecture. This contrast is virtually absent in the SRS channel, underscoring the high chemical selectivity of SRS.
4.4 Advantage of CRS Imaging
4.4.1 Speed Advantage
As discussed in Section 4.2 and Section 4.3, the image contrast generated by CRS is, in principle, no different from the vibrational contrast provided by spontaneous Raman techniques, yet the acquisition time for CRS is several orders of magnitude shorter. For this reason, CRS is ideal for high-throughput applications that demand fast acquisition times. A wide range of biological studies, from single-cell- to tissue-level studies, can benefit from faster acquisition rates. There are several practical advantages. First, a shorter acquisition time means that fewer photons are introduced to the sample, thus minimizing the possibility of photodamage. Second, arguably the most crucial advantage hinges on the fact that faster acquisition rates enable more measurements per time unit.
The latter advantage is crucial for biological imaging, particularly for studies that require recording a multitude of images in a short duration. For live tissue imaging, acquisition rates on the order of one image per second or faster is needed for capturing relevant dynamics in the tissue or for accounting for animal movement. Similarly, for tissue-level studies, the ability to image across multiple length scales is a desirable imaging property. Depending on the scope of the project, hundreds to thousands of high-resolution images – translating to over 108 pixels – may be required to characterize the distribution of molecules of interest. In practice, there are many applications that call for the generation of a large number of images in order to gain statistically meaningful data. For studies of this nature, pixel dwell times on the order of microseconds are a prerequisite. From this perspective, CRS stands out as an ideal molecular imaging tool because of its capability to rapidly generate high-resolution chemical maps, with imaging speed up to video rate (Figure 4.8) [12, 25].

Figure 4.8 Trade-off between acquisition speed and spectral coverage of Raman-based microscopies. The typical pixel dwell times for different modes of CRS imaging correlate inversely with the possible tissue coverage within a fixed duration (i.e., 1 h). It must be noted that the total acquisition time of the microscopy and microspectroscopy hybrid approach may progressively increase as the number of spectral points increases. Area highlighted in gray shows ideal pixel dwell time for live imaging and large tissue examination.
To best illustrate the advantages of CRS over spontaneous Raman system, let us consider a scenario where there are 10 000 points or 100 × 100 pixels of interest – a modest sampling number in the context of imaging. Using a typical spontaneous Raman setup with a dwell time of 1 s/pixel [26–28], approximately 2½ h would be needed to generate an image. Note that for larger image sizes the number of pixels increases quadratically and cubically with the number of images for 2D and 3D imaging, respectively. Hence, a million pixels correspond to merely 15 images with a moderate frame size of 256 × 256 pixels. Nevertheless, it would take 12 days to acquire such information with a typical spontaneous Raman system. When the contrast derived from one vibrational band is sufficient, then the same information can be realistically acquired within 1 s with a CRS microscope operating at 1 μs/pixel [12, 25]. It is this dramatic improvement in speed that has helped spur various applications of CRS in biology and medicine.
However, as mentioned before, the speed improvement does not come for free. In the next section, the trade-off between imaging speed and spectral information is discussed. Different modes of CRS imaging, including the pros and cons, are illustrated in context of relevant biological problems.
4.4.2 Speed versus Spectral Information
4.4.2.1 Single-Channel CRS Imaging
When a single vibrational band can be used to generate imaging contrast, CRS imaging offers unprecedented acquisition rates. CRS imaging speeds up to video rate with 30 frames per second have been reported [12, 25]. This mode of CRS imaging, although powerful for multifarious applications, is available at the cost of reduced spectral information. There are numerous cases where this sacrifice in chemical information is justifiable. For example, when the general composition of the sample is known, one vibrational mode may be sufficient to facilitate the visualization of the species of interest. By adjusting the CRS system to exclusively probe the CH2 symmetric stretch mode at ∼2850 cm−1, CH-rich molecules such as lipids can be visualized and discriminated from other molecules that contain fewer C–H bonds. In this manner, one can exploit the fast imaging speed of CRS to rapidly detect the presence and distribution of molecules of interest.
A study by Fu et al. illustrated the importance of combining molecular contrast with fast acquisition times for tissue imaging. They composed a high-resolution map of the entire mouse brain slice, allowing the visualization of cerebellar white matter, gray matter, corpus callosum, cortex, and fimbria hippocampus, based on the CH2 contrast from myelin, cellular membrane, lipid droplets, and other cellular structures present in brain [29]. A total of 9579 images were generated within 2.2 h to chemically map an ∼8 mm wide brain slice with submicrometer lateral resolution (Figure 4.9). Such a feat would be much more difficult to accomplish with spontaneous Raman system.

Figure 4.9 High acquisition speed of CRS enables chemical mapping of tissue at high spatial resolution. The whole mouse brain slice was constructed from 9579 CARS images that were acquired using a 20× objective, providing submicrometer lateral resolution. Various anatomical structures of the brain can be recognized based on the contrast from lipid-rich molecules present in myelin, cellular membrane, and neuronal cells. gcc, genu of corpus callosum; CPu, caudate putamen; ec, external capsule; dfi, dorsal fornix; fi, fimbria hippocampus; and LV, lateral ventricle.
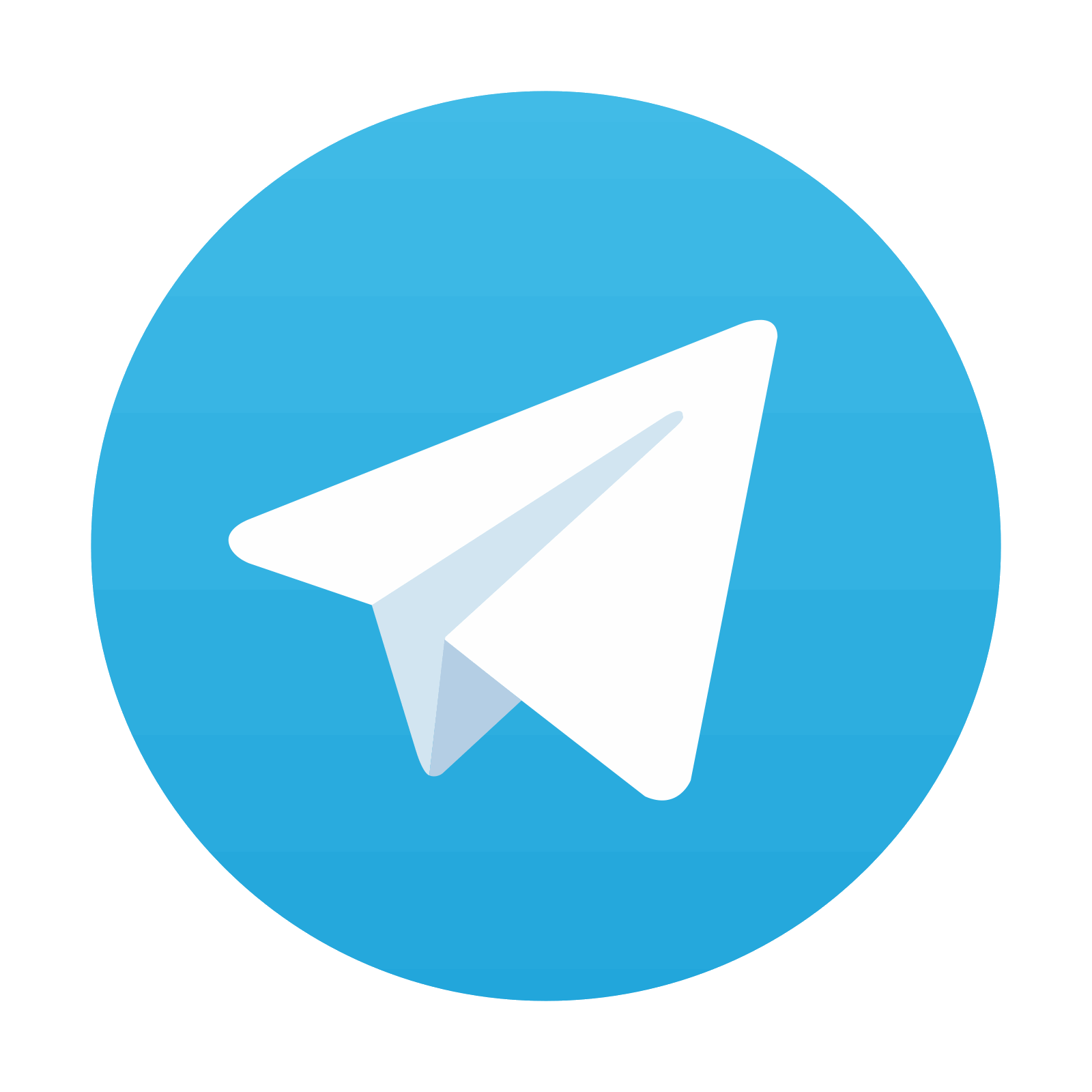
Stay updated, free articles. Join our Telegram channel
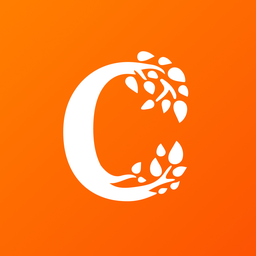
Full access? Get Clinical Tree
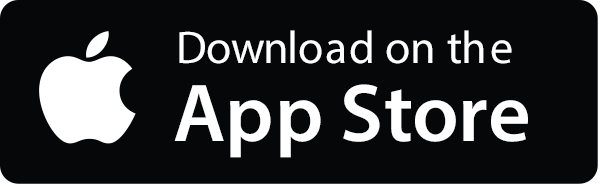
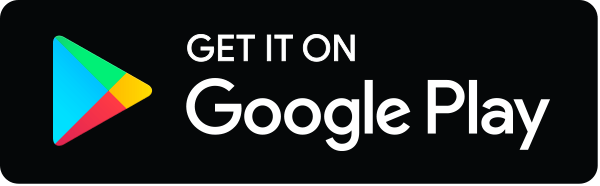