The previous two chapters have dealt with single-gene diseases. We turn now to diseases caused by alterations in the number or structure of chromosomes. The study of chromosomes and their abnormalities is called cytogenetics.
Chromosome abnormalities are responsible for a significant fraction of genetic diseases, occurring in approximately 1 of every 150 live births. They are the leading known cause of intellectual disability and pregnancy loss and an important cause of congenital malformations. Chromosome abnormalities are seen in 50% of first-trimester and 20% of second-trimester spontaneous abortions. Thus they are an important cause of morbidity and mortality.
As in other areas of medical genetics, advances in molecular genetics have contributed many new insights in the field of cytogenetics. For example, molecular techniques have permitted the identification of chromosome abnormalities, such as deletions (missing pieces of chromosome material), that affect regions as small as 10 kb. In many cases, specific genes that contribute to the phenotypes of cytogenetic syndromes are being pinpointed. In addition, the ability to identify DNA polymorphisms in parents and offspring has enabled researchers to specify whether an altered chromosome is derived from the mother or from the father. This has increased our understanding of the biological basis of meiotic errors and chromosome abnormalities.
In this chapter we discuss abnormalities of chromosome number and structure. The genetic basis of sex determination is reviewed, the role of chromosome alterations in cancer is examined, and several diseases caused by chromosomal instability are discussed. Emphasis is given to the recent contributions of molecular genetics to cytogenetics.
Cytogenetic Technology and Nomenclature
Although it was possible to visualize chromosomes under microscopes as early as the mid-1800s, it was quite difficult to observe individual chromosomes and thus to count the number of chromosomes in a cell or to examine structural abnormalities. Beginning in the 1950s several techniques were developed that improved our ability to characterize chromosomes. These included the use of spindle poisons such as colchicine and colcemid that arrest dividing somatic cells in metaphase, when chromosomes are maximally condensed and easiest to see; the use of a hypotonic (low-salt) solution, which causes swelling of cells, rupture of the nucleus, and better separation of individual chromosomes; and the use of staining materials that are absorbed differently by different parts of chromosomes, producing the characteristic light and dark bands that help to identify individual chromosomes.
Our ability to study chromosomes has been improved by the visualization of chromosomes in metaphase, by hypotonic solutions that cause nuclear swelling, and by staining techniques that label chromosome bands.
Chromosomes are typically analyzed by collecting a living tissue (usually blood), culturing the tissue for the appropriate amount of time (usually 48 to 72 hours for peripheral lymphocytes), adding colcemid to produce metaphase arrest, harvesting the cells, placing the cell sediment on a slide, rupturing the cell nucleus with a hypotonic saline solution, staining with a designated nuclear stain, and photographing the metaphase spreads of chromosomes on the slide. The images of the 22 pairs of autosomes are arranged according to length, with the sex chromosomes in the right-hand corner. This ordered display of chromosomes is termed a karyogram or karyotype ( Fig. 6.1 ). (The term karyotype refers to the number and type of chromosomes present in an individual, and karyogram is now often used to designate the printed display of chromosomes.) Currently, computerized image analyzers are used to display chromosomes.

After sorting by size, chromosomes are further classified according to the position of the centromere . If the centromere occurs near the middle of the chromosome, the chromosome is said to be metacentric ( Fig. 6.2 ). An acrocentric chromosome has its centromere near the tip, and submetacentric chromosomes have centromeres somewhere between the middle and the tip. The tip of each chromosome is the telomere. The short arm of a chromosome is labeled p , and the long arm is labeled q. In metacentric chromosomes, where the arms are of roughly equal length, the p and q arms are designated by convention.
A karyotype, or karyogram, is a display of chromosomes ordered according to length. Depending on the position of the centromere, a chromosome may be acrocentric, submetacentric, or metacentric.

A normal female karyotype is designated 46,XX; a normal male karyotype is designated 46,XY. The nomenclature for various chromosome abnormalities is summarized in Table 6.1 and is indicated for each condition discussed in this chapter.
Karyotype | Description |
---|---|
46,XY | Normal male chromosome constitution |
47,XX,+21 | Female with trisomy 21, Down syndrome |
47,XY,+21[10]/46,XY[10] | Male who is a mosaic of trisomy 21 cells and normal cells (10 cells scored for each karyotype) |
46,XY,del(4)(p15) | Male with distal and terminal deletion of the short arm of chromosome 4 from band p15 to terminus |
46,XX,dup(5)(p14p15.3) | Female with a duplication within the short arm of chromosome 5 from bands p14 to p15.3 |
45,XY,der(13;14)(q10;q10) | A male with a balanced Robertsonian translocation of chromosomes 13 and 14; karyotype shows that one normal 13 and one normal 14 are missing and replaced with a derivative chromosome composed of the long arms of chromosomes 13 and 14 |
46,XY,t(11;22)(q23;q22) | A male with a balanced reciprocal translocation between chromosomes 11 and 22; the breakpoints are at 11q23 and 22q22 |
46,XX,inv(3)(p21q13) | An inversion on chromosome 3 that extends from p21 to q13; because it includes the centromere, this is a pericentric inversion |
46,X,r(X)(p22.3q28) | A female with one normal X chromosome and one ring X chromosome formed by breakage at bands p22.3 and q28 with subsequent fusion |
46,X,i(Xq) | A female with one normal X chromosome and an isochromosome of the long arm of the X chromosome |
Chromosome Banding
Early karyotypes were useful in counting the number of chromosomes, but structural abnormalities, such as balanced rearrangements or small chromosomal deletions, were often undetectable. Staining techniques were developed in the 1970s to produce the chromosome bands characteristic of modern karyotypes. Chromosome banding helps greatly in the detection of deletions, duplications, and other structural abnormalities, and it facilitates the correct identification of individual chromosomes. The major bands on each chromosome are systematically numbered ( Fig. 6.3 ). For example, 14q32 refers to the second band in the third region of the long arm of chromosome 14. Subbands are designated by decimal points following the band number (e.g., 14q32.3 is the third subband of band 2).

Several chromosome-banding techniques are used in cytogenetics laboratories. Quinacrine banding (Q-banding) was the first staining method used to produce specific banding patterns. This method requires a fluorescence microscope and is no longer as widely used as Giemsa banding (G-banding). To produce G-bands, a Giemsa stain is applied after the chromosomal proteins are partially digested by trypsin. Reverse banding (R-banding) requires heat treatment and reverses the usual white and black pattern that is seen in G-bands and Q-bands. This method is particularly helpful for staining the distal ends of chromosomes. Other staining techniques include C-banding and nucleolar organizing region stains (NOR stains). These latter methods specifically stain certain portions of the chromosome. C-banding stains the constitutive heterochromatin, which usually lies near the centromere, and NOR staining highlights the satellites and stalks of acrocentric chromosomes (see Fig. 6.2 ). C-banding and NOR staining are useful in identifying unknown chromosomal material.
High-resolution banding involves staining chromosomes during prophase or early metaphase (prometaphase) before they reach maximal condensation. Because prophase and prometaphase chromosomes are more extended than metaphase chromosomes, the number of bands observable for all chromosomes increases from about 300 to 450 (as in Fig. 6.3 ) to as many as 800. This allows the detection of less obvious abnormalities usually not seen with conventional banding.
Chromosome bands help to identify individual chromosomes and structural abnormalities in chromosomes. Banding techniques include quinacrine, Giemsa, reverse, C, and NOR banding. High-resolution banding, using prophase or prometaphase chromosomes, increases the number of observable bands.
Fluorescence in Situ Hybridization
In the widely used fluorescence in situ hybridization (FISH) technique, a labeled single-stranded DNA segment (probe) is exposed to denatured metaphase, prophase, or interphase chromosomes from a patient. The probe undergoes complementary base pairing (hybridization) only with the complementary DNA sequence at a specific location on one of the denatured chromosomes. Because the probe is labeled with a fluorescent dye, the location at which it hybridizes with the patient’s chromosomes can be visualized under a fluorescence microscope. A common use of FISH is to determine whether a portion of a chromosome is deleted in a patient. In an unaffected person, a probe hybridizes in two places, reflecting the presence of two homologous chromosomes in a somatic cell nucleus. If a probe from the chromosome segment in question hybridizes to only one of the patient’s chromosomes, then the patient probably has the deletion on the copy of the chromosome to which the probe fails to hybridize. FISH provides considerably better resolution than high-resolution banding approaches and can typically detect deletions as small as 1 million base pairs (1 Mb) in size. It is widely used to detect common deletion syndromes such as Prader–Willi syndrome (microdeletion of 15q11.2) and Williams syndrome (microdeletion of 7q11.2) (discussed later).
Extra copies of a chromosome region can also be detected using FISH. In this case the probe hybridizes in three or more places instead of two. Combinations of FISH probes can also be used to detect chromosome rearrangements such as translocations (see later discussion).
Fig. 6.4A illustrates a FISH result for a child who is missing a small piece of the short arm of chromosome 17. Although a centromere probe (used as a control) hybridizes to both copies of chromosome 17, a probe corresponding to a specific region of 17p hybridizes to only one chromosome. This demonstrates the 17p11.2 deletion that causes the Smith–Magenis syndrome (see Fig. 6.4B ; also see Table 6.3 later in the Chapter).

Abnormality | Prevalence at Birth |
---|---|
Autosomal Syndromes | |
Trisomy 21 | 1/700 |
Trisomy 18 | 1/6000 |
Trisomy 13 | 1/10,000 |
Unbalanced rearrangements | 1/17,000 |
Balanced rearrangements | |
| 1/1000 |
| 1/11,000 |
Sex Chromosome Abnormalities | |
47,XXY | 1/1000 male births |
47,XYY | 1/1000 male births |
45,X ∗ | 1/5000 female births |
47,XXX | 1/1000 female births |
All Chromosome Abnormalities | |
Autosomal disorders and unbalanced rearrangements | 1/230 |
Balanced rearrangements | 1/500 |
∗ The 45,X karyotype accounts for about half of the cases of Turner syndrome.
Syndrome | Clinical Features | Chromosomal Deletion |
---|---|---|
Prader–Willi | Intellectual disability, short stature, obesity, hypotonia, characteristic facies, small feet | 15q11–13 |
Angelman | Intellectual disability, ataxia, uncontrolled laughter, seizures | 15q11–13 |
Langer–Giedion | Characteristic facies, sparse hair, exostosis, variable intellectual disability | 8q24 |
Miller–Dieker | Lissencephaly, characteristic facies, significant cognitive and psychomotor disability | 17p13.3 |
Velocardiofacial/DiGeorge | Characteristic facies, cleft palate, heart defects, poorly developed thymus | 22q11 |
Smith–Magenis | Intellectual disability, hyperactivity, dysmorphic features, self-destructive behavior | 17p11.2 |
Williams | Developmental disability, characteristic facies, supravalvular aortic stenosis | 7q1 |
Aniridia, Wilms tumor | Intellectual disability, predisposition to Wilms tumor, genital defects | 11p13 |
Deletion 1p36 | Intellectual disability, seizures, hearing loss, heart defects, growth failure, distinctive facial features | 1p36 |
Rubinstein–Taybi | Intellectual disability, broad thumbs and great toes, characteristic facial features, vertebral and sternal abnormalities, heart defects | 16p13.3 |
Alagille | Neonatal jaundice, “butterfly” vertebrae, pulmonic valvular stenosis, characteristic facial features | 20p12 |
∗ For most of these conditions, only some cases are caused by the listed microdeletion; other cases may be caused by single-gene mutations within the same region.
Because FISH detection of missing or extra chromosomes can be carried out with interphase chromosomes, it is not necessary to stimulate cells to divide to obtain metaphase chromosomes (a time-consuming procedure required for traditional microscopic approaches). This allows analyses and diagnoses to be completed more rapidly. FISH analysis of interphase chromosomes is commonly used in the prenatal detection of fetal chromosome abnormalities and in the analysis of chromosome rearrangements in tumor cells.
The FISH technique has been extended by using multiple probes, each labeled with a different color, so that several of the most common numerical abnormalities (e.g., those of chromosomes 13, 18, 21, X, and Y) can be tested simultaneously in the same cell. In addition, techniques such as spectral karyotyping use varying combinations of five different fluorescent probes in conjunction with special cameras and image-processing software so that each chromosome is uniquely colored (painted along its entire length with a series of same-colored probes) for ready identification. Such images can be especially useful for identifying small chromosome rearrangements ( Fig. 6.5 ). With the increasing use of techniques such as comparative genomic hybridization (see below), spectral karyotyping is now rarely needed in clinical practice.
FISH is a technique in which a labeled probe is hybridized to metaphase, prophase, or interphase chromosomes. FISH can be used to test for missing or additional chromosomal material as well as chromosome rearrangements. The FISH technique can be extended with multiple colors to detect several possible alterations of chromosome number simultaneously. Multiple probes can be used to paint each chromosome with a unique color, facilitating the detection of structural rearrangements.

Comparative Genomic Hybridization and Cytogenomic Microarrays
Losses or duplications of whole chromosomes or specific chromosome regions can be detected by a widely used technique known as comparative genomic hybridization (CGH) ( Fig. 6.6 ). DNA is extracted from a test source such as cells from a tumor or whole-blood cells from a patient. The DNA is then labeled with a substance that exhibits one color (e.g., red) under a fluorescence microscope; DNA taken from normal control cells is labeled with a second color (e.g., green). In the earliest version of CGH, both sets of DNA are then hybridized to normal metaphase chromosomes on a slide. If any chromosome region is duplicated in the tumor cell, then the corresponding region of the metaphase chromosome will hybridize with excess quantities of the red-labeled DNA. This region will appear red under the microscope. Conversely, if a region is deleted in the tumor cell, then the corresponding region of the metaphase chromosome will hybridize only with green-labeled control DNA, and the region will appear green under the microscope. CGH is especially useful in scanning for deletions and duplications of chromosome material in cancer cells, where detection of such alterations can help to predict the type and/or severity of the cancer.

A serious limitation of CGH when used with metaphase chromosomes is that deletions or duplications smaller than 5 to 10 Mb cannot be detected microscopically. Higher resolution is offered by array CGH (aCGH), in which test and control DNA is hybridized with a microarray that contains hundreds of thousands to millions of oligonucleotide probes (see Chapter 3 ) whose DNA sequences correspond to specific regions of the genome. These microarrays provide resolution to 50 to 100 kb or even less, allowing the detection of duplications and deletions ( copy number variants ) that may affect only a single gene.
More recently microarrays have utilized single nucleotide polymorphisms (SNPs; see Chapter 3 ) arranged even closer together and allowing for detection of deletions and duplications of less than 20 kb in size ( Fig. 6.7 ). This newer genomics-based technology is termed cytogenomic microarray (CMA) and now the preferred approach in the clinical laboratory setting. CMA also can detect loss of heterozygosity (see Chapter 11 ) and uniparental disomy, adding more sensitivity and a wider range of diagnostic yield.

Array CGH and CMA offer a number of other advantages over traditional karyotype analysis. The process is highly automated, requiring less time from laboratory personnel. There is no need for dividing cells (in contrast to the analysis of metaphase chromosomes), and less than a microgram of DNA is sufficient for analysis of the entire genome. For these reasons, aCGH and CMA have become the most commonly used techniques in cytogenetics laboratories. The primary disadvantage of these techniques is that they cannot detect balanced rearrangements of chromosomes (i.e., reciprocal translocations or inversions) because the quantity of chromosome material remains unchanged.
The CGH technique, in which differentially labeled DNA from test and control sources is hybridized to probes in microarrays, allows the detection of chromosome duplications and deletions. CMA offers higher resolution than CGH and can detect duplications, deletions, uniparental disomy, and loss of heterozygosity. Array CGH and CMA can detect deletions and duplications much smaller than standard karyotyping and require only small amounts of DNA, but they cannot detect balanced rearrangements of chromosomes.
Abnormalities of Chromosome Number
Polyploidy
A cell that contains a multiple of 23 chromosomes in its nucleus is said to be euploid (Greek, eu = “good,” ploid = “set”). Thus haploid gametes and diploid somatic cells are euploid. Polyploidy, the presence of a complete set of extra chromosomes in a cell, is seen commonly in plants and often improves their agricultural value. Polyploidy also occurs in humans, although much less frequently. The polyploid conditions that have been observed in humans are triploidy (69 chromosomes in the nucleus of each cell) and tetraploidy (92 chromosomes in each cell nucleus). The karyotypes for these two conditions are designated 69,XXX and 92,XXXX, respectively (assuming that all of the sex chromosomes were X; other combinations of the X and Y chromosomes may be seen). Because the number of chromosomes present in each of these conditions is a multiple of 23, the cells are euploid in each case. However, the additional chromosomes encode a large amount of surplus gene product, causing multiple anomalies such as defects of the heart and central nervous system.
Triploidy is seen in about 1 in 10,000 live births, but it accounts for an estimated 15% of the chromosome abnormalities occurring at conception. The vast majority of triploid conceptions are spontaneously aborted, and this condition is one of the most common causes of fetal loss in the first two trimesters of pregnancy. Triploid fetuses that survive to term typically die shortly after birth. The most common cause of triploidy is the fertilization of an egg by two sperm (dispermy). The resulting zygote receives 23 chromosomes from the egg and 23 chromosomes from each of the two sperm cells. Triploidy can also be caused by the fusion of an ovum and a polar body, each containing 23 chromosomes, and subsequent fertilization by a sperm cell. Meiotic failure, in which a diploid sperm or egg cell is produced, can also produce a triploid zygote.
Tetraploidy is much rarer than triploidy, both at conception and among live births. It has been recorded in only a few live births, and those infants survived for only a short period. Tetraploidy can be caused by a mitotic failure in the early embryo; all of the duplicated chromosomes migrate to one of the two daughter cells. It can also result from the fusion of two diploid zygotes.
Cells that have a multiple of 23 chromosomes are said to be euploid. Triploidy (69 chromosomes) and tetraploidy (92 chromosomes) are polyploid conditions found in humans. Most polyploid conceptions are spontaneously aborted, and all are incompatible with long-term survival.
Autosomal Aneuploidy
Cells that contain missing or additional individual chromosomes are termed aneuploid (not a multiple of 23 chromosomes). Usually only one chromosome is affected, but it is possible for more than one chromosome to be missing or duplicated. Aneuploidies of the autosomes are among the most clinically important of the chromosome abnormalities. They consist primarily of monosomy (the presence of only one copy of a chromosome in an otherwise diploid cell) and trisomy (three copies of a chromosome). Autosomal monosomies are nearly always incompatible with survival to term, so only a small number of them have been observed among live-born individuals. In contrast, some trisomies are seen with appreciable frequencies among live births. The fact that trisomies produce less-severe consequences than monosomies illustrates an important principle: the body can tolerate excess genetic material more readily than it can tolerate a deficit of genetic material.
The most common cause of aneuploidy is nondisjunction, the failure of chromosomes to disjoin normally during meiosis ( Fig. 6.8 ). Nondisjunction can occur during meiosis I or meiosis II (see Chapter 2 ). The resulting gamete either lacks a chromosome or has two copies of it, producing a monosomic or trisomic zygote, respectively.
Aneuploid conditions consist primarily of monosomies and trisomies. They are usually caused by nondisjunction. Autosomal monosomies are almost always lethal, but some autosomal trisomies are compatible with survival.

Trisomy 21
Trisomy 21 (karyotype 47,XY,+21 or 47,XX,+21) ∗ is seen in approximately 1 of every 700 to 1000 live births, making it the most common autosomal aneuploid condition compatible with survival to term. This trisomy is the most common cause of Down syndrome, a phenotype originally described by John Langdon Down in 1866. Almost 100 years elapsed between Down’s description of this syndrome and the discovery (in 1959) that it is caused by the presence of an extra copy of chromosome 21.
∗ For brevity, the remainder of the karyotype designations for abnormalities not involving the sex chromosomes will indicate an affected male.
Although there is considerable variation in the appearance of persons with Down syndrome, they present with a constellation of features that help the clinician to make a clinical diagnosis. The facial features include a depressed nasal root, upslanting palpebral fissures, measurably small and sometimes overfolded ears, and a flattened maxillary and malar region, giving the face a characteristic appearance ( Fig. 6.9 ). The cheeks are round, and the corners of the mouth are sometimes downturned. Some of these features led to the use of the term “mongolism” in earlier literature, but this term is inappropriate and pejorative and is no longer used. The neck is short, and the skin is redundant at the nape of the neck, especially in newborns. The occiput is flat, and the hands and feet tend to be rather broad and short. Approximately 50% of persons with Down syndrome have a single flexion crease across their palms (single palmar crease, formerly termed a “simian crease,” though this term is also now considered inappropriate). Decreased muscle tone (hypotonia) is a highly consistent feature that is also helpful in making a diagnosis. None of these features are diagnostic per se as all are observed in the general population of infants; it is the clustering together that suggests the diagnosis.

Several medically significant problems occur with increased frequency among infants and children with Down syndrome. About 3% of these infants develop an obstruction of the duodenum or atresia (closure or absence) of the esophagus, duodenum, or anus. Respiratory infections are quite common, and the risk of developing leukemia is 15 to 20 times higher in patients with Down syndrome than in the general population. The most significant medical problem is that approximately 40% of these individuals are born with structural heart defects. The most common of these is an atrioventricular (AV) canal, a defect in which the interatrial and interventricular septa fail to fuse normally during fetal development. The result is blood flow from the left side of the heart to the right side and then to the pulmonary vasculature, producing pulmonary hypertension. Ventricular septal defects (VSDs) are also common.
Moderate to mild intellectual disability † (standardized IQ ranging from 40 to 60) occurs in most persons with Down syndrome, and this condition alone accounts for approximately 10% of all cases of intellectual disability in the United States. In recent years clinical trials designed to test whether specific medications might improve attention and learning in school age-children with Down syndrome have been initiated (see https://www.clinicaltrials.gov ).
† Because of the potentially stigmatizing aspects of the term “mental retardation,” many professional organizations and advocacy groups related to Down syndrome currently have abandoned the term and use intellectual or cognitive disability in its place.
Several other medical problems occur in infants and young children with Down syndrome. Conductive and sometimes sensorineural hearing loss, hypothyroidism, and various eye abnormalities are the most important and common. Clinical Commentary 6.1 outlines a plan for routine medical care of infants and children with Down syndrome.
An approach termed health supervision and anticipatory guidance has evolved for the care and treatment of persons with genetic syndromes and chronic diseases. After a thorough study of the disease in question (including an extensive literature review), basic guidelines are established for the screening, evaluation, and management of patients. If followed by the primary care practitioner or the specialist, these guidelines should help to prevent further disability or illness. We illustrate the health supervision and anticipatory guidance approach with the current guidelines for care of children with Down syndrome.
- ▪
As mentioned in the text, AV canals are the most common congenital heart defect seen in newborns with Down syndrome. Surgical correction of this condition is appropriate if it is detected before 1 year of age; after this time, pulmonary hypertension has been present too long for surgery to be successful. Accordingly, it is now recommended that an echocardiogram be performed during the newborn period and no later than 6 months.
- ▪
Because Down syndrome patients often have strabismus (deviation of the eye from its normal visual axis), congenital cataracts, and other eye problems, they should be examined regularly by their physician. Because of the frequency of eye manifestations, the current guidelines of the American Academy of Pediatrics (AAP) recommend referral to an ophthalmologist familiar with Down syndrome during the first 6 months of life. In asymptomatic children, an ophthalmological examination before the age of 4 years is recommended to evaluate visual acuity.
- ▪
In the primary care setting growth curves calculated specifically from individuals with Down syndrome can be used in following growth and determining when a child has growth deficiency.
- ▪
Hypothyroidism is common, especially during adolescence. First confirm that the newborn screening for thyroid disease is normal, and then thyroid hormone levels should be measured annually starting at one year of age.
- ▪
Sensorineural and conductive hearing loss are both seen in children with Down syndrome. The routine follow-up should include a hearing test at birth and every 6 months until 2 years of age, with subsequent testing as needed.
- ▪
Obstructive sleep apnea (OSA) is very common in persons with Down syndrome of all ages. Clinicians should have a high index of suspicion for OSA; the AAP guidelines recommend referral to a sleep disorder clinic or pulmonologist for a polysomnogram (sleep study) before 4 years of age.
- ▪
Instability of the first and second vertebrae has led to spinal cord injuries in some older Down syndrome patients. It is thus suggested that imaging studies be carried out in children with neurological symptoms and in those planning to participate in athletic activities.
- ▪
Referral of infants and children with Down syndrome to preschool programs to provide intervention for developmental disabilities is an important component of routine care.
- ▪
Refer families to a support group and provide written information and Internet resources.
Similar series of guidelines have been developed for children with trisomy 18, Williams syndrome, Marfan syndrome, and Turner syndrome. In principle, the anticipatory guidance and health supervision approach can be applied to any genetic disease for which there is sufficient knowledge of natural history. The GeneReviews resource and the text “Management of Genetic Syndromes” as well as the AAP guidelines (see Suggested Reading) provide expert opinion and sometimes consensus guidelines for routine care of persons with genetic conditions.
The medical problems seen in children with Down syndrome can result in decreased survival rates. Congenital heart defects are the most important single cause of early mortality. In the early 1960s only about half of children with Down syndrome survived as long as 5 years. As a result of improvements in corrective surgery, antibiotic treatment, and management of leukemia, the survival rate has increased considerably in the past 50 years. Currently it is estimated that more than 90% of children with Down syndrome will survive to age 5 years, and the majority survive past 50 years of age. Over 97% of children without serious structural heart defects will reach their fifth birthday, as will 92% with heart disease, due to advances in surgery and early treatment. Additionally, there is convincing evidence that enriched environments and educational interventions can produce significant improvements in intellectual function. Overall survival and medical health has improved significantly in the last four decades, dramatically altering the quality of life of persons with Down syndrome.
Males with Down syndrome are nearly always sterile, with only a few reported cases of reproduction. Many females with Down syndrome can reproduce, although approximately 40% fail to ovulate. A female with Down syndrome has a 50% risk of producing a gamete with two copies of chromosome 21 (which would then produce a trisomic zygote). However, because about 75% of trisomy 21 conceptions are spontaneously aborted, the risk of producing affected live-born offspring is considerably lower than 50% in women with Down syndrome. Because reproduction is uncommon, nearly all cases of trisomy 21 can be regarded as new mutations.
Approximately 95% of Down syndrome cases are caused by nondisjunction (see Fig 6.7 ), and most of the remainder are caused by chromosome translocations (see later discussion). Comparisons of chromosome 21 polymorphisms in parents and offspring have demonstrated that the extra chromosome is contributed by the mother in 90% to 95% of trisomy 21 cases. About 75% of these maternal nondisjunctions occur during meiosis I, and the remainder occur during meiosis II. As discussed in greater detail later, there is a strong correlation between maternal age and the risk of producing a child with Down syndrome.
Mosaicism (see Chapter 4 ) is seen in approximately 2% to 4% of trisomy 21 live births. These persons have some normal somatic cells and some cells with trisomy 21. This type of mosaicism in a male is designated 47,XY,+21[10]/46,XY[10], with the numbers inside the brackets indicating the number of cells scored with each karyotype. The most common cause of mosaicism in trisomy is a trisomic conception followed by loss of the extra chromosome during mitosis in some embryonic cells. Mosaicism often results in milder clinical expression of the phenotype associated with a chromosome abnormality.
Depending on the timing and the way the mosaicism originated, some persons have tissue-specific mosaicism. As the term suggests, this type of mosaicism is confined only to certain tissues. This can complicate diagnosis because cytogenetic analysis is usually based on a single tissue type (usually circulating lymphocytes derived from a blood sample, or less commonly, fibroblasts derived from a skin biopsy). Mosaicism affecting primarily the germline of a parent can lead to multiple recurrences of Down syndrome in the offspring. This factor helps to account for the fact that the recurrence risk for Down syndrome among mothers younger than 30 years is about 1% (i.e., 10 times higher than the population risk for this age group).
Because of the prevalence and clinical importance of Down syndrome, considerable effort has been devoted to determining which genes on chromosome 21 account for the features of this disorder. A candidate for intellectual disability in Down syndrome is DYRK1A, a kinase gene that causes learning and memory defects when it is overexpressed in mice. Another gene located in the critical region, APP, encodes the amyloid β precursor protein. A third copy of APP is likely to account for the occurrence of Alzheimer disease features in nearly all Down syndrome patients by 40 years of age. Mutations of APP cause a small percentage of Alzheimer disease cases (see Chapter 12 ), and Down syndrome individuals with partial trisomies that do not include the APP gene do not develop Alzheimer disease features.
Trisomy 21, which causes Down syndrome, is the most common autosomal aneuploidy seen among live births. The most significant features include intellectual disability, gastrointestinal tract obstruction, congenital heart defects, and respiratory infections. The extra 21st chromosome is contributed by the mother in approximately 90% of cases. Mosaicism is seen in 2% to 4% of Down syndrome cases, and it often accompanies a milder phenotype. Specific genes contributing to the Down syndrome phenotype are being identified.
Trisomy 18
Trisomy 18 (47,XY,+18), also known as Edwards syndrome, is the second most common autosomal trisomy, with a prevalence of about 1 per 6000 live births. However, it is much more common at conception and is the most common chromosome abnormality among stillborns with congenital malformations. It is estimated that fewer than 5% of trisomy 18 conceptions survive to term.
The Edwards syndrome phenotype is as discernible as Down syndrome, but because it is less common, it is less likely to be recognized clinically. Infants with trisomy 18 have prenatal growth deficiency (weight that is low for gestational age), characteristic facial features, and a distinctive hand abnormality that often helps the clinician to make the initial diagnosis ( Fig. 6.10 ). Minor anomalies of diagnostic importance include small ears with unraveled helices, a small mouth that is often hard to open, a short sternum, and short halluces (first toes). Most babies with trisomy 18 have major malformations. Congenital heart defects, particularly VSDs with dysplasia of multiple heart valves, are the most common and occur in 90% of children. Other medically significant congenital malformations include omphalocele (protrusion of the bowel into the umbilical cord), trachea-esophageal fistula with esophageal atresia, radial aplasia (missing radius bone), diaphragmatic hernia, and occasionally spina bifida.

About 50% of infants with trisomy 18 die within the first few weeks of life, and about 12% survive to 12 months of age. Mortality figures from population studies performed in the last three decades showed only 5% to 8% survival in the first year. But recent large-scale investigations from the US and Canada have demonstrated increased survival and suggested that a shift toward more technological intervention improves outcome. A combination of factors, including aspiration pneumonia, predisposition to infections, central apnea, upper airway obstruction, and congenital heart defects, accounts for the high mortality rate. Notably if a child with trisomy 18 does survive to one year, the chance that the infant will live to past age 5 years is quite high, indicating that those who survive to 12 months likely represent a selected group.
Marked developmental disabilities are seen among those trisomy 18 patients who survive to infancy. The degree of psychomotor delay is much more significant than in Down syndrome, and most children are not able to walk independently. However, children with trisomy 18 do progress in their milestones, albeit slowly, and older children learn some communication skills. Recent studies report that some older children have walked unassisted.
More than 95% of patients with Edwards syndrome have complete trisomy 18; only a small percentage have partial 18q trisomy or mosaicism. As in trisomy 21, there is a significant maternal age effect, and more than 95% of trisomy 18 cases are the result of an extra chromosome transmitted by the mother.
Trisomy 13
Trisomy 13 (47,XY,+13), also termed Patau syndrome, is seen in about 1 of every 10,000 births. The malformation pattern is quite distinctive and usually permits clinical recognition. It consists primarily of orofacial clefts, microphthalmia (small, abnormally formed eyes), and postaxial polydactyly ( Fig. 6.11 ). Malformations of the central nervous system are often seen, as are heart defects (80%) and renal abnormalities. Cutis aplasia (a defect of the skin of the scalp on the posterior occiput) can also occur and be helpful as a diagnostic clue.
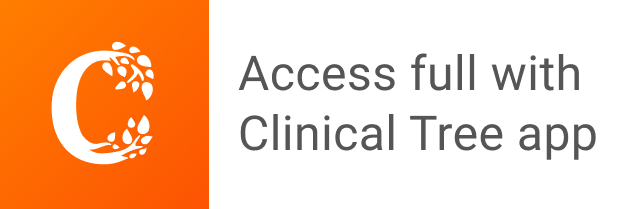