Paediatrics
In this section, we discuss the following areas of diagnosis:
Specimen collection from neonates and children
This should be done expertly, and the amount of blood taken minimised. Capillary blood is used in neonatal units and for newborn screening. Blood specimens from babies are obtained using an automated neonatal device by heel-prick from the fleshy (lateral) parts of the heel. In older children, finger-prick capillary sampling is used to monitor diabetes.
Pediatric reference ranges
Age-related reference ranges must always be used. In neonates, particularly when premature or small for gestational age, interpretation of results is especially difficult and requires considerable experience.
Causes and diagnosis of biochemical disturbances in the neonate and early childhood
Glucose
Neonates
The foetus utilises maternal glucose and any excess is stored as hepatic glycogen. Fat is also stored in foetal adipose tissue during the last trimester. After birth the baby has to maintain its own blood glucose between feeds by fat and glycogen catabolism and gluconeogenesis. In some babies these mechanisms are insufficient to maintain blood [glucose], and hypoglycaemia occurs. In the neonate a blood [glucose] less than 2.5 mmol/L is widely regarded as being an intervention threshold. The principal causes of neonatal hypoglycaemia are as follows (see Table 21.1):
Table 21.1 Infants at risk of developing hypoglycaemia
Poor milk intake | Hormone imbalance |
Establishment of breastfeeding | Hyperinsulinaemia |
Decreased glycogen stores | Growth hormone |
Prematurity | deficiency |
Small for gestational age | Adrenal insufficiency |
Some inborn errors | Hypopituitarism |
Illness | |
Increased demand | |
Sepsis | |
Hypoxia, hypothermia and pyrexia | |
Inborn errors |
- Transient hypoglycaemia in healthy term infants is most commonly due to a low milk intake during establishment of breastfeeding. Premature infants, and infants that are small for gestational age, are particularly at risk from hypoglycaemia due to their lack of glycogen and fat stores.
- Hypoglycaemia may also become evident if there is an increased demand for glucose such as may occur in the septic, hypoxic, hypothermic or pyrexial infant.
- In rare cases the hypoglycaemia may be due to an inherited metabolic disorder or a deficiency or imbalance in a range of hormones including GH and glucocorticoids.
Hyperinsulinaemia is the most common cause of persistent or recurrent neonatal hypoglycaemia after the first 24 h of life. There are a number of causes including maternal hyperglycaemia due to poor diabetic control during pregnancy. Maternal hyperglycaemia induces hyperplasia of foetal islet cells, resulting in foetal hyperinsulinaemia that cannot be suppressed in the neonate, and hypoglycaemia results. Rare genetic causes of persistent hyperinsulinism have been identified including deficiencies of glucokinase or glutamate dehydrogenase or recessive mutations of the genes for the sulphonylurea receptor (SUR 1) and the ATP- dependent potassium channel (KIR6.2) in the plasma membrane of the pancreatic β cells. The gene defects in SUR 1 and KIR 6.2 give rise to hyperplasia of insulin-producing cells in the pancreas, a condition known as ‘nesidioblastosis’. Sporadic causes (e.g. Beckwith syndrome) have also been identified. Neonates at risk of hypoglycaemia should have their blood glucose monitored regularly by POCT, with low readings (<2.5 mmol/L) being confirmed by blood collection for laboratory measurement before treatment.
During childhood
Recurrent hypoglycaemia of infancy and childhood may be due to any of the causes listed in Table 6.5 (p. 100) as well as several other inherited metabolic disorders (e.g. fatty acid oxidation disorders and mitochondrial disorders). Although nesidioblastosis usually presents in the first few days of life, symptoms may be delayed for up to 6 months.
Calcium and magnesium
Plasma [calcium] falls, in normal full-term infants, by about 10–20% in the first 2–3 days of life. It then returns to normal (2.0–2.8 mmol/L) over the course of the next 3–4 days.
Neonatal hypocalcaemia within the first 48 h, sufficient to give rise to twitching, irritability and convulsions, occurs particularly in infants who are premature, those of diabetic mothers and those who have experienced birth asphyxia. Maternal vitamin D deficiency may be a factor (e.g. in Asian women). The mechanism is complex, but the hypocalcaemia tendency usually corrects spontaneously, although calcium gluconate may need to be given if convulsions occur, or if plasma [calcium] falls to less than 1.50 mmol/L. Rarely, hypocalcaemic convulsions in the neonate are associated with maternal hyperparathyroidism, which may produce temporary hypoparathyroidism in the neonate due to suppression of the foetal parathyroid glands by maternal hypercalcaemia.
Late neonatal hypocalcaemia, between the fourth and 10th days of life, may occur in full-term as well as in premature infants. Hyperexcitability of muscles is usually also present. This is liable to occur in infants whose mothers had a low intake of vitamin D during pregnancy; these infants may also have low plasma [magnesium]. Rarely, hypocalcaemia may be due to renal failure. Treatment with calcium, and often magnesium, may be required.
Neonatal rickets. Premature infants have increased requirements for calcium, phosphate and vitamin D for bone growth. The most sensitive indicator of inadequate intake is a rising plasma ALP, which precedes abnormalities in plasma calcium or phosphate.
Hypomagnesaemia is an occasional cause of neonatal convulsions. It can lead to hypocalcaemia by reducing PTH secretion. Plasma [magnesium] should be measured in all cases of hypocalcaemia.
Hypercalcaemia in the neonatal period is less common than hypocalcaemia. Inadequate phosphate supplements in rapidly growing pre-term infants can lead to hypophosphataemia, bone resorption and thus hypercalcaemia. Oversupplementation may also lead to hypercalcaemia. Much rarer causes include immobilisation, malignancy, hyperparathyroidism, familial hypocalciuric hypercalcaemia and William’s syndrome.
Neonatal jaundice (Table 21.2)
Physiological unconjugated hyperbilirubinaemia
Approximately half of all infants develop hyperbilirubinaemia during the first week of life due to
- Increased production from breakdown of red cells, which have a shortened lifespan in the neonate.
- Decreased excretion, because of immaturity of hepatic bilirubin uptake, conjugation and biliary excretion.
- Reabsorption from the intestine. Bilirubin is deconjugated by the intestinal enzyme β-glucuronidase. In adults it is further metabolised to urobilinogen by normal intestinal bacteria. The newborn’s GI tract has not yet acquired these organisms, and some bilirubin is re-absorbed, a process which is stimulated by breast milk.
Table 21.2 Causes of jaundice in the neonatal period
Early jaundice | Prolonged jaundice |
Physiological | Breastfeeding |
Haemolysis | Prematurity |
Infection | Infection |
Genetic defects of bilirubin metabolism | Biliary atresia Endocrine disorders Genetic disorders |
Pathological causes of unconjugated hyperbilirubinaemia
Babies may be at increased risk of severe hyperbili-rubinaemia if they have one or more of the following, in addition to normal physiological factors.
- Dehydration, intercurrent infection, bruising, polycythaemia.
- Increased haemolysis
- Lysis due to Rhesus incompatibility is now a rare occurrence; however, lysis may also result from ABO or other blood group incompatibility
- Predisposition to lysis due to glucose-6-phosphate dehydrogenase deficiency, hereditary spherocytosis.
- Lysis due to Rhesus incompatibility is now a rare occurrence; however, lysis may also result from ABO or other blood group incompatibility
- Defective hepatic uptake or conjugation. This may occur in association with Gilbert’s syndrome, mutations in anion transporters or rarely inherited disorders of bilirubin metabolism (Crigler–Najjar syndrome, p. 203).
- Variation in the concentration of circulating proteins (particularly albumin) that bind unconjugated bilirubin.
- Displacement of bilirubin from protein-binding sites by drugs (e.g. benzyl penicillin, phenobarbital, furosemide), free fatty acids (hypoglycaemic and ketotic infants) or hydrogen ions (acidotic infants).
- Impairment of the blood-brain barrier in premature and sick infants, allowing some albumin-bound bilirubin to penetrate the brain.
Total plasma bilirubin measurement is used routinely as a proxy for free bilirubin. First-line screen for healthy term babies is by visual assessment or transcutaneous bilirubin monitor. Confirmation requires laboratory measurement of total plasma bilirubin. Circulating unconjugated bilirubin is removed by phototherapy or, if concentrations are grossly elevated, by exchange transfusion. Action limits for treatment to prevent kernicterus are age related and are lower for preterm or sick infants.
Prolonged jaundice is defined as jaundice persisting beyond 14 days of age. It is most commonly breast milk jaundice, mainly due to increased enterohepatic circulation, and caused by unconjugated bilirubin alone. It does not usually require treatment. All prolonged jaundice requires measurement of total and conjugated (or direct) bilirubin.
Conjugated hyperbilirubinaemia
Any increase in conjugated (which may be measured as direct) bilirubin always indicates pathology and requires identification and further investigation of its aetiology by appropriate chemical and other tests.
Causes include infective, endocrine and genetic disorders (Table 21.3).
Biliary atresia. Extra-hepatic biliary atresia is a rapidly progressing condition where the bile ducts are rapidly obliterated. Diagnosis is confirmed by ultrasound scan to demonstrate absence of the gall bladder. Surgery before 60 days of age may be curative, otherwise the only treatment option is liver transplant. To identify potentially life-threatening but treatable causes, it is imperative to investigate all cases of prolonged jaundice without delay, by measurement of total and conjugated bilirubin, and liver function tests in the first instance. Further tests such as thyroid function, urine reducing substances (for galactosaemia) and biliary tract imaging are carried out after clinical assessment of the infant.
Table 21.3 Causes of conjugated hyperbilirubinaemia in the neonate
Biliary atresia | Cystic fibrosis |
Sepsis or neonatal hepatitis | Galactosaemia |
Hypothyroidism | Tyrosinaemia |
Hypopituitarism (±septo–optic dysplasia) | α1-Anti-trypsin deficiency |
Inherited metabolic disorders
Inborn errors of metabolism usually present in infancy or childhood. They result from alteration in a single gene, leading to a protein product that has suboptimal function. Most of these rare conditions show autonomic recessive inheritance: heterozygotes do not manifest the disorder. The affected protein may be an enzyme, a structural or transport protein or an enzyme cofactor affecting the activity of several enzymes (Table 21.4).
The consequences of an inherited defect affecting a metabolic pathway include:
- Accumulation of potentially toxic metabolites that occur in the pathway before the defect.
- Deficiency of essential metabolites produced by the pathway after the defect.
- I ncreased flux of potentially toxic metabolites through alternative metabolic pathways.
- Storage of macromolecules in organs such as liver and spleen if the defect is in their breakdown pathway.
- Negative feedback inhibition of the pathway activity may fail because production of the end-product is decreased.
Figures 21.1–21.3 illustrate these effects in PKU, defects in fatty acid oxidation and galactosaemia, respectively. The age at presentation depends on residual protein activity and is not constant for a given disorder.
In some cases addition or deletion of chromosomes or parts of chromosomes occur, leading to clinically significant abnormalities (e.g. Down’s syndrome, Turner’s syndrome).
Table 21.4 Examples of inherited metabolic disorders
General disorders | Examples |
Amino acid metabolism | Phenylketonuria, tyrosinaemia |
Organic acid metabolism | Methylmalonic aciduria |
Fatty acid oxidation | Medium chain acyl–CoA dehydrogenase deficiency |
Urea cycle defects | Ornithine transcarbamylase deficiency |
Carbohydrate disorders | Galactosaemia |
Defects of intermediary metabolism | Mitochondrial respiratory chain defects |
Steroid synthesis defects | 21–Hydroxylase, 11–hydroxylase deficiencies |
Macromolecule breakdown defects | Lysosomal storage disorders |
Transport protein defects | Cystic fibrosis |
Cofactor defects | Biotinidase deficiency |
Organelle assembly or uptake | Peroxisomal defects |
Diagnosis
The individual disorders are rare, but collectively they are not uncommon. The true incidence is unknown, except for those that are detected by screening programmes. In acute neonatal presentations, an infant assessed as normal at birth typically develops reluctance to feed, vomiting and abnormal breathing. Without treatment the affected infants rapidly progress to multiple organ failure, coma and death. A second group may have a more chronic and progressive course, with symptoms such as failure to thrive, progressive hepatomegaly or neurological deterioration developing over months or years. Several inherited metabolic disorders that present less acutely may nevertheless carry a very poor prognosis. Only a minority present with a clearly defined clinical syndrome.
For the index case in a family, the recognition that there is a metabolic disease present, and its identification, can present complex diagnostic problems.
The index case
In an acutely ill infant, or one presenting with chronic signs and symptoms, the results of routine laboratory tests may provide a clue to a potential inborn error of metabolism. These include positive urine reducing substances, metabolic acidosis identified by blood gas analyser or calculated anion gap, hypoglycaemia or haematological abnormalities such as neutropenia, haemolytic anaemia, megaloblastic anaemia and coagulation defects. Suggestive clinical features include dys-morphia, hepato- or splenomegaly, cardiomyopathy, neurological disorders, intractable feeding difficulties or onset of acute symptoms in response to fasting or weaning. Depending on the specific presentation, further blood testing by the local laboratory may include some of the following: liver function tests including coagulation screen, calcium, creatinine, urea, urate, glucose, lactate, urate, cholesterol, potassium, sodium, chloride and ammonia.
Samples may be referred to a regional paediatric laboratory for
- Urine, plasma or CSF amino acids
- Urine organic acids
- Galactosaemia screening test (red cell galactose-1-phosphate uridylyltransferase)
- Bloodspot acyl carnitine profile
- Mucopolysaccharide excretion
- Specific enzyme tests (to diagnose, for example, lysosomal storage disorders or mitochondrial respiratory chain defects).
For the correct interpretation of results it is essential to provide clinical details including fed/fasted status (fasting potentiates abnormalities in fatty acid oxidation and gluconeogenetic defects), transfusion history (any transfused red cells invalidate the galactosaemia screening test) and drug therapy (antibiotics mask amino acid abnormalities) at the time of specimen collection.
Confirmation of the diagnosis
Diagnostic confirmation may require tissue biopsy (skin, muscle, liver) to demonstrate deficient enzyme activity or a pathological DNA mutation. Specific therapy (e.g. enzyme replacement therapy for Gaucher’s disease, biotin supplements in bio-tinidase deficiency) is available for some disorders. Even if no such therapy is available, precise diagnosis is essential to allow genetic counselling for the family, including investigation of siblings and other relatives, genetic advice on recurrence rates and potential pre-natal diagnosis during future pregnancies.
Fetal tissue can be obtained by the technique of chorionic villus biopsy for the purpose of pre-natal diagnosis. These techniques have been applied to a wide variety of diseases including the diagnosis of haemoglobinopathies, cystic fibrosis (p. 300) and Duchenne muscular dystrophy (p. 182). Heterozygote detection is not usually reliable by enzyme analysis, and is now done by mutation studies. It is particularly important for female relatives of boys diagnosed with an X-linked disorder (e.g. ornithine transcarbamylase deficiency, MPS II).
Examples of inherited metabolic disorders
Phenylketonuria (PKU)
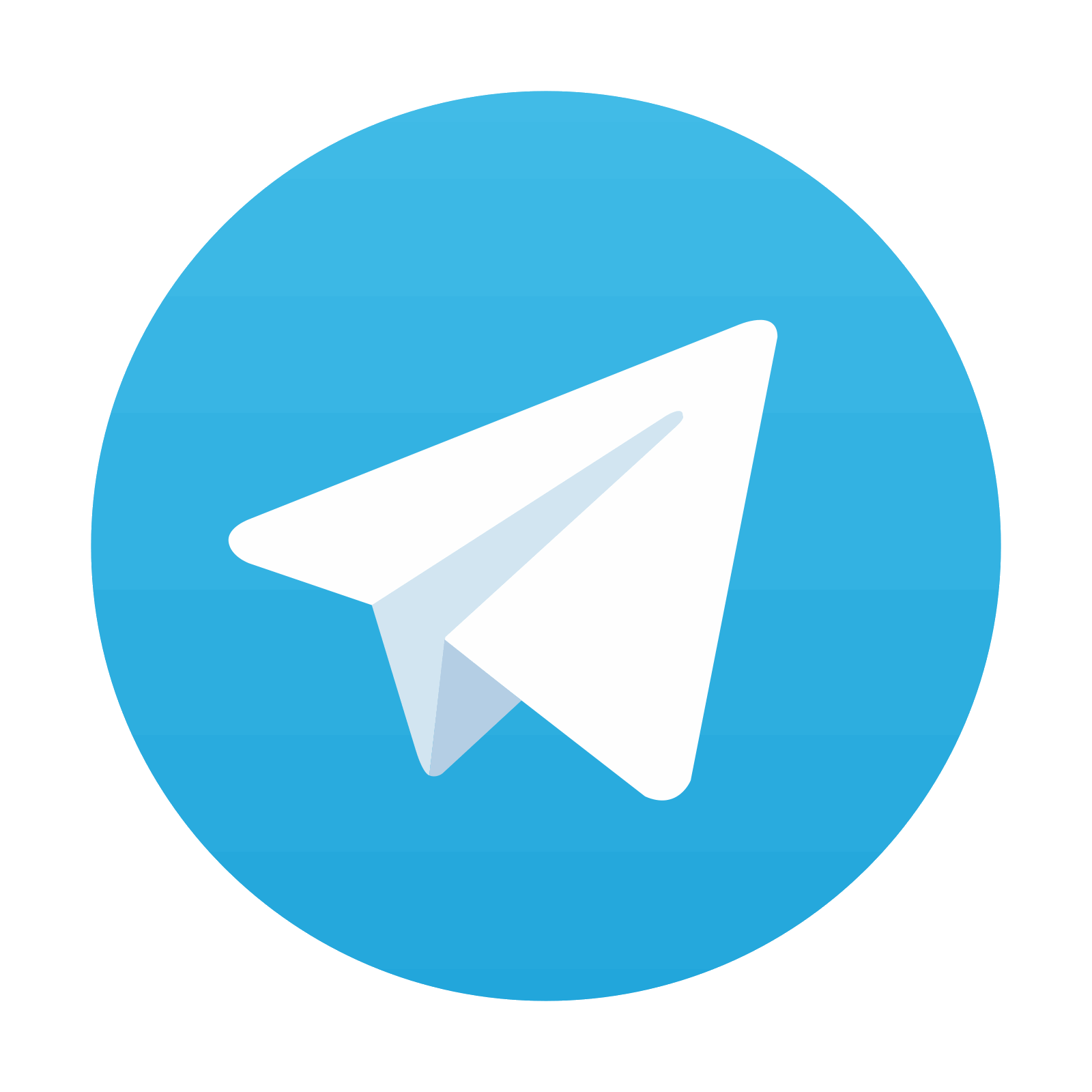
Stay updated, free articles. Join our Telegram channel
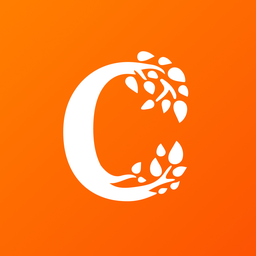
Full access? Get Clinical Tree
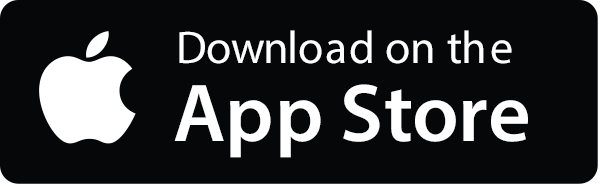
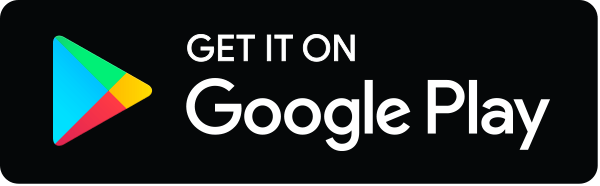