Overview
The breakdown (catabolism) and synthesis (anabolism) of carbohydrate molecules represent the primary means for the human body to store and utilize energy and to provide building blocks for molecules such as nucleotides (Figure 6-1). The enzyme reactions that form the metabolic pathways for monosaccharide carbohydrates (Chapter 2) include glycolysis, the citric acid cycle, and oxidative phosphorylation as the main means to produce the energy molecule adenosine triphosphate (ATP). Gluconeogenesis and the pentose phosphate pathway represent the two main anabolic pathways to produce new carbohydrate molecules. Glycogen has its own metabolic pathway for lengthening, shortening, and/or adding branch points in the carbohydrate chain(s). Not surprisingly, all of these processes are highly regulated at multiple points to allow the human body to efficiently utilize these important biomolecules. Finally, many modified carbohydrates are part of a variety of surface and cytosolic signaling molecules, including glycoproteins and glycosaminoglycans (GAGs) (Chapter 2). These important carbohydrate molecules and the control points in carbohydrate and glycoprotein metabolism, therefore, present clinicians with opportunities to modify these many reactions to improve health or to fight disease.
Figure 6-1.
Overview of Carbohydrate Metabolism. Glucose from the diet can be metabolized via glycolysis or glycogenesis. Resulting metabolic products can return to glucose via gluconeogenesis or glycogenolysis, respectively, or proceed further along carbohydrate metabolism to the citric acid cycle. Alternatively, glucose products can be shunted off to fat or amino acid metabolism as indicated. Details are discussed in the text and other chapters. [Adapted with permission from Murray RA, et al.: Harper’s Illustrated Biochemistry, 28th edition, McGraw-Hill, 2009.]
Glycolysis
Glycolysis is the metabolic pathway that breaks down (catabolism) hexose (six-carbon) monosaccharides such as glucose, fructose, and galactose into two molecules of pyruvate, two molecules of ATP, two molecules of NADH, two water (H2O) molecules, and two hydrogen ions (H+) (Figure 6-2). Glycolysis involves 10 enzyme-mediated steps and is best envisioned in two phases—phosphorylation and energy production—all of which occur in the cytoplasm. The phosphorylation phase (sometimes referred to as the preparatory phase) starts with the six-carbon carbohydrate glucose and involves two phosphorylations from ATP and the cleavage into two molecules of the trisaccharide (three-carbon sugar) glyceraldehyde-3-phosphate. The energy production phase involves the next five steps during which the two molecules of glyceraldehyde-3-phosphate are converted to two pyruvate molecules with the production of two NADH molecules and four ATP molecules. Glucose-6-phosphate, the first intermediate of glycolysis, cannot exit the cell-like glucose, so it also traps the glucose molecule in the cell for energy production via glycolysis or glycogen synthesis (see below). NADH represents an alternative energy storage form than ATP, which may be utilized by the oxidative phosphorylation pathway.
Figure 6-2.
Glycolysis. The pathway of glycolysis includes 10 enzyme steps, which break down one molecule of glucose to two molecules of pyruvate, yielding two ATP and two NADH molecules. Pyruvate subsequently enters the mitochondria and the citric acid cycle (see below). Glycolysis can be divided into phosphorylation (Steps 1–5) and energy production (Steps 6–10) phases for better comprehension. Enzymes are indicated by yellow-shaded boxes. [Adapted with permission from Naik P: Biochemistry, 3rd edition, Jaypee Brothers Medical Publishers (P) Ltd., 2009.]
Not surprisingly, all three regulatory steps involve either an investment or production of ATP molecules, a committing process in the balance of energy production and storage. The first reaction of glycolysis (catalyzed by hexokinase) results in the addition of a phosphate group to form the molecule glucose-6-phosphate. In the liver and pancreas, hexokinase is replaced by the enzyme glucokinase. Phosphofructokinase-1 adds a second phosphate group to produce fructose-1,6-bisphosphate. Pyruvate kinase, the final reaction in glycolysis, produces an ATP molecule. Although not regulated at the enzyme level such as the above enzymes, phosphoglycerate kinase is involved in the initial production of ATP from glycolysis and represents the “break even” point when ATP invested in the preparation phase is gained back. Although regulated in many different ways and by many different molecules, a theme emerges that, when energy stores are low, glycolysis is activated to produce more ATP. When energy levels are high, either the glycolytic pathway is inhibited or alternative pathways are promoted for storage of the glucose molecule for later use. Regulation of carbohydrate metabolism and these important regulatory enzymes will be discussed in further detail in later chapters (e.g., Chapter 10).
Phosphofructokinase Deficiency: Because of its importance in energy production, diseases involving enzymes from glycolysis are rare. One example, though, is the deficiency of phosphofructokinase, also known as glycogen storage disease type VII or Tarui’s disease. It is inherited in an autosomal recessive manner. This disease leads to a buildup of glycogen in cells and impairs various red blood and muscle cells from using glucose and many other monosaccharides to produce ATP. There are three different variations of the disease, depending on the severity of impairment. The first, termed “infantile,” presents in the first year of life usually starting with blindness/cataracts and retardation with death by the age of 4 years. A second “classic” variant presents in childhood with muscle cramping and weakness after exercise and death/breakdown of muscle cells as well as low red blood cell count (anemia). The third “late onset” variant presents in early adulthood with progressive weakness of arms and legs but without muscle cramping/breakdown as well as anemia. Diagnosis is by noting high levels of glycogen in muscle samples and measuring the activity of phosphofructokinase. The only treatment is avoidance of high carbohydrate meals and any vigorous exercise. |
ATP is the primary energy molecule used by the human body (Figure 6-3). Three enzymes involved in glycolysis, hexokinase, phosphofructokinase-1, and pyruvate kinase, are regulated to allow the body to decide both whether to use its carbohydrate resources for ATP production and how much to produce. At each step, the cell decides to continue energy production or to channel the resulting intermediates into other molecules such as glycogen, lipids, and/or proteins. The ability of the human body to sense its biochemical surroundings and then respond to that environment enables intelligent and efficient use of its limited resources.
Figure 6-3.
ATP Structure with Its Magnesium Cofactor. ATP serves as the primary energy molecule of the human body utilizing the energy contained in the phosphate bonds. Magnesium (Mg2+) stabilizes these bonds and hence is necessary for ATP to be biologically functional. [Reproduced with permission from Murray RA, et al.: Harper’s Illustrated Biochemistry, 28th edition, McGraw-Hill, 2009.]
Citric Acid Cycle
The citric acid cycle is the second metabolic pathway involved in the catabolism of carbohydrates into energy and involves eight enzyme steps, starting and ending with the molecule oxaloacetate (Figure 6-4). In humans, all of the steps of the citric acid cycle, including the linking reaction of pyruvate conversion to acetyl-CoA, take place within the mitochondrial matrix, the area within the inner mitochondrial membrane. Pyruvate enters the citric acid cycle following its conversion to acetyl-CoA; the reaction is catalyzed by pyruvate dehydrogenase that links glycolysis to the citric acid cycle and produces one molecule of NADH. By means of acetyl-CoA and intermediates in this cycle, the body can also divert fats and amino acids into energy production (discussed later in this chapter). In addition, the citric acid cycle provides precursor molecules for the synthesis of amino acids and/or receives these amino acids when proteins are used for energy production (see Chapter 5, Figure 5-7).
Figure 6-4.
Citric Acid Cycle. The citric acid cycle includes eight enzymatic steps (tan-shaded boxes), which receive pyruvate from glycolysis via the acetyl-CoA molecule. The cycle produces one GTP, three NADH, and one FADH2 as it progresses to oxaloacetate at which point the cycle repeats. [Adapted with permission from Murray RA, et al.: Harper’s Illustrated Biochemistry, 28th edition, McGraw-Hill, 2009.]
Acetyl-CoA, a two-carbon molecule bonds to the four-carbon molecule oxaloacetate to form citrate, the namesake of this pathway. This pathway is also known as the tricarboxylic acid cycle, because citrate contains three carboxylic acid groups, or the Krebs cycle after the physician/biochemist Sir Hans Krebs, who earned a Nobel Prize for his work on elucidating this sequence of reactions. Further enzyme reactions remove carbon molecules in the form of carbon dioxide (CO2) and H2O. By doing so, the cycle produces three molecules of NADH, one molecule of FADH2, and one molecule of guanosine triphosphate (GTP), all of which may be used in energy production and storage by oxidative phosphorylation (see below) and conversion to ATP.
The regulation of the citric acid cycle is mainly via the availability of each enzyme’s substrate as well as inhibition when the concentration of any enzyme’s product gets too high. One prime example is citrate, the product of the first reaction, which inhibits not only the cycle but also phosphofructokinase activity in glycolysis. That citrate is the first intermediate of the cycle and is important in the control of the body’s carbohydrate resources. As with glycolysis, the same general rules also apply: if energy levels (in this case NADH or FADH2, which are converted to ATP via the later oxidative phosphorylation pathway) are high, the citric acid cycle is slowed or intermediates are diverted to other purposes.
Red Blood Cells (Erythrocytes) and Glycolysis: Red blood cells are unique in the human body in that they lose their nuclei and organelles, including mitochondria, early in their development (Chapter 14). As a result, red blood cells are unable to utilize the citric acid cycle or oxidative phosphorylation and are solely reliant on ATP production from glycolysis. NADH produced from glycolysis is used to produce lactate from pyruvate; the resulting lactate diffuses from the red blood cells and is transported into and used by tissues such as heart muscle and liver. In addition, red blood cells do not respond to insulin like other tissues in the human body and are, therefore, not regulated by this important hormonal signal that controls carbohydrate metabolism. |
Oxidative Phosphorylation
The third and final pathway of conversion of carbohydrates to energy is oxidative phosphorylation (Figure 6-5). This metabolic pathway takes place exclusively inside the inner mitochondrial membrane, taking advantage of differences in concentration of the products of the citric acid cycle to drive the formation of ATP.
Figure 6-5.
Overview of Oxidative Phosphorylation. Electrons are accepted from NADH (Complex I), succinate (Complex II), reduced Q protein via Complex II-generated FADH2 (Complex III), and from cytochrome c (Complex IV). The resulting excess of hydrogen drives ATPase synthase (Complex V) to produce ATP. The process uses molecular oxygen (O2) and the final product of this series of reactions is water (H2O). [Adapted with permission from Murray RA, et al.: Harper’s Illustrated Biochemistry, 28th edition, McGraw-Hill, 2009.]
Specifically, oxidative phosphorylation includes both the respiratory chain (i.e., oxidative) and ATP synthesis via ATP synthase [i.e., phosphorylation of adenosine diphosphate (ADP)]. The respiratory chain portion involves four complexes (complexes I–IV) and a Q protein that help to transport H+ from the inside of the mitochondria to the intermembrane space between the inner and outer membranes. These H+ ions can then readily diffuse to the cytoplasm so that the pH in the intermembrane space and the cytoplasm become equivalent. Complex II is actually the same enzyme seen in the citric acid cycle (succinate dehydrogenase; Figure 6-4) and is responsible for the generation of one FADH2 and a molecule of fumarate for each molecule of succinate. Complex I accepts electrons from NADH, complex II accepts electrons from succinate, and complex III accepts electrons from reduced Q protein that itself receives electrons from FADH2 generated from complex II. Complex IV accepts electrons from cytochrome c molecules (see Figure 6-5). The process uses molecular oxygen (O2) and the final product of this series of reactions is H2O.
The four complexes are thought to be associated into a “super” complex, allowing the H+ to be channeled from one complex to another; if this concept is correct, oxidative phosphorylation illustrates how association of several enzymes together can increase their efficiency, a theme that will be repeated several times in human biochemistry. ATP synthase (also called complex V) then transports the H+ back through the inner membrane into the matrix, utilizing the energy from the H+ concentration gradient to form ATP (Figure 6-5).
Toxins and Poisons—Blocking Oxidative Phosphorylation: Because oxidative phosphorylation represents a key step in the production of biological energy, it is also the target of various chemical agents. Cyanide and carbon monoxide, both toxic substances, interfere with hemoglobin molecules but also specifically inhibit complex IV, blocking the respiratory chain. Tissues highly dependent on energy production (e.g., nervous and heart muscles) are particularly affected, resulting in headache, dizziness, seizures, coma, and cardiac arrest leading to death. Antidotes (e.g., nitrites and sodium thiosulfate) work by causing the release of complex IV from the cyanide molecule followed by metabolism of the nontoxic product. Some insecticides (e.g., rotenone) also work via blocking specific complexes of oxidative phosphorylation. |
NADH is often produced outside the mitochondrial matrix
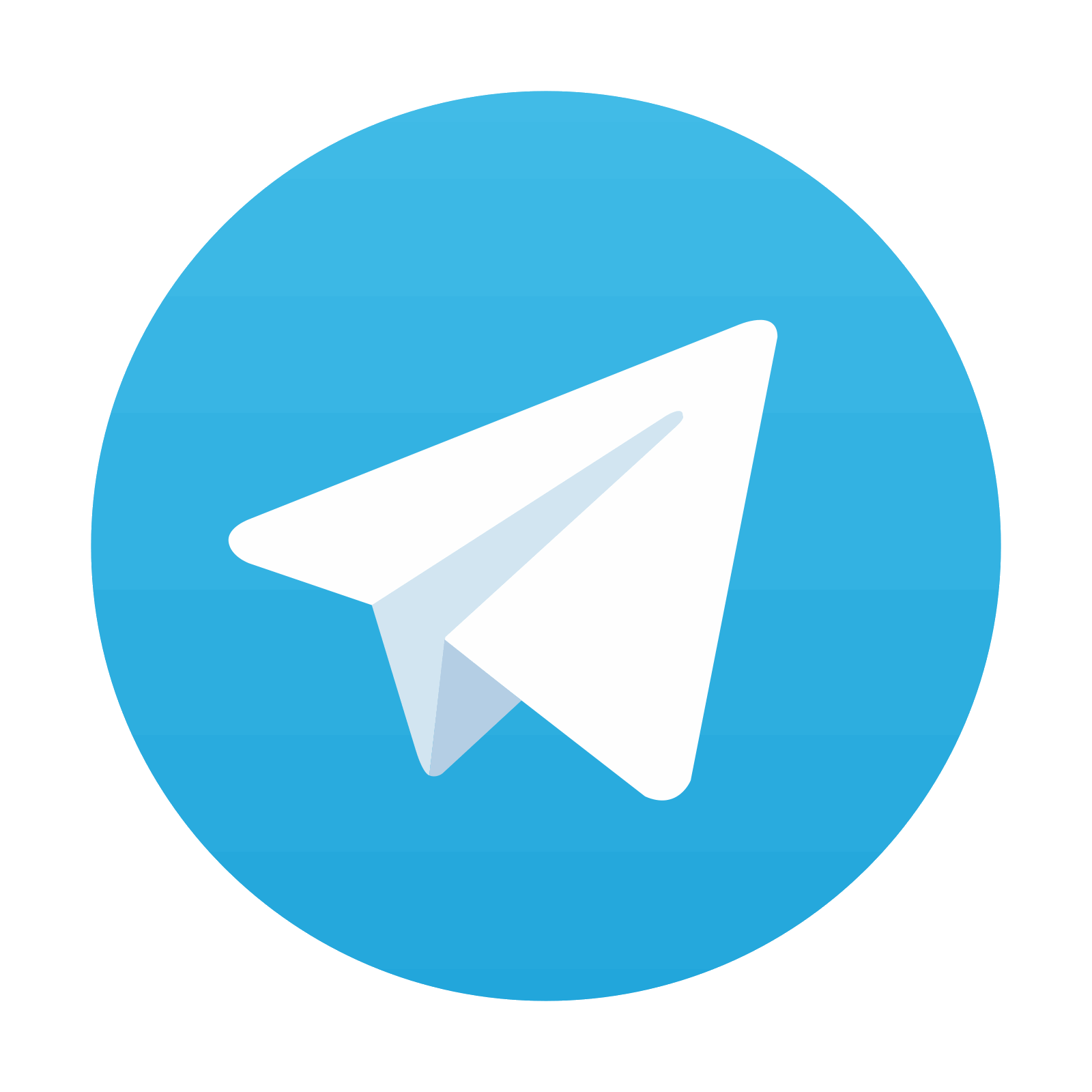
Stay updated, free articles. Join our Telegram channel
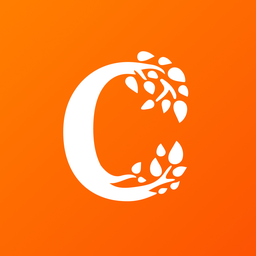
Full access? Get Clinical Tree
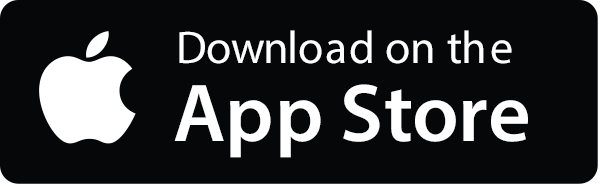
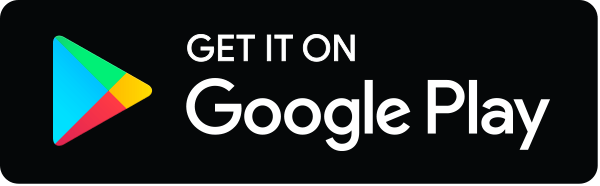
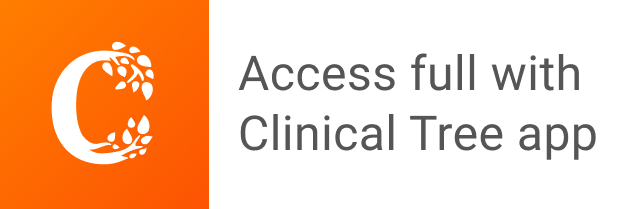