Overview
Blood, including red cells, white cells, platelets, and a collection of specialized proteins, serves an essential physiologic function as it carries molecules from one part of the body to another. This chapter discusses the biochemical underpinnings of how blood transports oxygen from the lungs and iron to the tissues that need them without causing damage to the tissues that do not. This transport system also insures adequate waste removal from the human body. In addition, the biochemical properties of clotting, the system that maintains the integrity of the blood vasculature, are discussed. In describing the normal way in which blood carries out these functions, the discussion will illustrate, from a biochemical perspective, the ways diseases may result when these processes go awry.
These three important roles, however, are just a small fraction of the critical functions that blood carries out to maintain the proper performance of the human body. The core biochemical principles of these three systems—the role of allostery in regulating ligand binding in the oxygen–hemoglobin system, the specialized functions of proteins for iron transport and storage, and the importance of biochemical cascades as illustrated by the clotting system—apply broadly to other functions of the blood and other organ systems in general. Thus, by understanding these fundamental biochemical mechanisms, a general foundation for understanding the normal physiology and pathophysiology of other systems can be established.
Basic Components of Blood
Hematology, the study of blood, has been of central importance in medicine throughout history. For much of history, health and illness were considered a reflection of different “humors” in the body: black bile, yellow bile, phlegm, and blood. Good health was attained when all the humors were in harmonic balance and illness was considered as the result of an imbalance in the humors. Thus, the goal of healers was to restore the balance of the humors in those who were ill. To manipulate blood, healers employed methods such as applying leeches, cupping, and bloodletting in hope of achieving health through creating proper balance of humors.
A human adult contains approximately 5 liters (l) of blood, with the entire volume circulating through the body every 1–2 min. Centrifugation separates blood into a cellular layer, which collects at the bottom end of the collection tube, and a noncellular layer, located at the top of the tube (Figure 14-1). If whole blood clots before centrifugation, the remaining noncellular component is called serum. On the other hand, if anticlotting additives are added to the test tube, the noncellular layer is called plasma. The cellular and noncellular layer each account for approximately 50% of the total blood volume.
Figure 14-1.
Separation of Blood into its Basic Components. Upon centrifugation, blood separates into a noncellular “plasma” layer at the top of the tube; a middle “buffy coat,” composed of platelets and white blood cells; and a bottom layer of mostly red blood cells. Because this sample contains anticlotting additives, the noncellular layer is called plasma. [Reproduced with permission from Mescher AL: Junqueira’s Basic Histology Text and Atlas, 12th edition, McGraw-Hill, 2010.]
The cellular compartment consists of red blood cells (RBCs), the most abundant cell in the body, white blood cells, and platelets. The generation of new RBCs (erythrocytes) from bone marrow is termed erythropoiesis, and it relies on the kidney-secreted hormone erythropoietin (Epo) (see below). White blood cells, which primarily serve to fight infections, can be further divided into specific subtypes and are discussed in Chapter 15. Platelets are small cells that primarily participate in clotting. The clotting system consists of both cellular and noncellular components and will be discussed below. Similar to the cellular layer, the noncellular layer can be divided into various components, each of which carries out essential functions for maintaining health. For instance, within the noncellular component are carrier proteins, which transport other proteins or small molecules. Important examples include hemoglobin (Hgb), which carries oxygen (O2) and carbon dioxide (CO2), and transferrin, which transports iron and regulates iron metabolism. Other carrier proteins transport small peptides, lipophilic hormones (Chapter 3), and even drugs.
The most abundant noncellular protein in the blood is albumin (3.5–5 g/dl). Albumin is secreted by liver cells (hepatocytes, discussed in Chapter 11), serves as a carrier protein, and, most importantly, serves to maintain oncotic pressure. Oncotic pressure is the term used to describe the force that keeps fluid from leaking out of a container through a diffusible barrier. When albumin levels become low (hypoalbuminemia), such as with liver failure (inadequate albumin is made) or in protein-losing diseases (where too much albumin is lost through either urine or stool), the oncotic pressure of the blood falls. This change in pressure causes noncellular fluid to leak from the blood into the tissues, resulting in edema (swelling). Edema can be life-threatening, particularly pulmonary edema, where lungs become fluid filled and O2 exchange is impaired. Pulmonary edema from pure hypoalbuminemia is extremely rare, and the most common causes of pulmonary edema are heart failure and inflammation. Immunoglobulins (antibodies) form the second most common type of protein in the blood (2.3–3.5 g/dl). Although immunoglobulins play a minor role in maintaining blood oncotic pressure, the most serious complication from low immunoglobulin levels is an increased risk of infection (Chapter 15). The blood also transports hormones, small bioactive molecules that affect the function of one or multiple target organs and tissues (Chapters 1 and 3). In addition to protein components, serum contains an incredible variety of nonprotein components, including minerals, electrolytes, lipoprotein particles, and essential nutrients (such as glucose and glutamine).
Red Blood Cell (RBC) Functions
RBCs are small cells (6–8 μm in diameter) whose primary function is to transport O2 from the lungs to the peripheral tissues. A secondary function of RBCs is to carry CO2, generated by the peripheral tissues, back to the lungs for elimination by exhalation (Chapter 17) through the mouth and nose. Hgb is the O2-carrying molecule in RBCs. Hgb consists of four heme molecules with four globin chains, giving the molecule its name. Adult Hgb is a tetramer of four globin chains: two α-globin subunits and two β-globin subunits (Figure 14-2A–C and 14-3A–B).
Figure 14-2.
A–C. Basic Components of a Red Blood Cell. (A) Each erythrocyte (red blood cell) contains many molecules of (B) hemoglobin (Hgb), a four-subunit molecule. Each subunit of Hgb contains (C) one heme molecule. [Adapted with permission from Murray RA, et al.: Harper’s Illustrated Biochemistry, 28th edition, McGraw-Hill, 2009.]
Figure 14-3.
A–B. Synthesis and Structure of Hemoglobin (Hgb). (A) Heme is synthesized via multiple enzymatic steps (indicated in blue), which occur in both the mitochondria and cytosol. Increased levels of heme negatively regulate ALA synthase, the first enzyme in the synthetic pathway. The final step, catalyzed by ferrochelatase, is responsible for the addition of Fe2+ to the finished heme molecule. Defective enzymes in all of the remaining synthetic steps lead to a series of diseases noted in red. (B) A heme molecule consists of a protoporphyrin ring with a centrally suspended iron molecule (see the text below). This iron atom binds oxygen (O2); therefore, one Hgb molecule can bind a maximum of four O2 molecules. CoA, coenzyme A; PLP, pyridoxal phosphate. [Adapted with permission from Naik P: Biochemistry, 3rd edition, Jaypee Brothers Medical Publishers (P) Ltd., 2009.]
Each heme molecule consists of two parts: a protoporphyrin molecule and iron. The local environment created by each globin subunit maintains iron in the ferrous (Fe2+) state. In this form, iron has six “coordination sites” that interact with the protoporphyrin ring and other molecules. The first four of the coordination sites bind to the protoporphyrin ring along its plane, suspending the iron in the middle of the ring (Figure 14-4A). The fifth site forms a strong, covalent linkage to Hgb through histidine (called the “proximal histidine”) of the globin chain (Figure 14-4A). Sites 1 through 5 are fixed. The sixth site, however, is not fixed and accounts for the versatility of Hgb binding. It is the sixth site that binds and releases O2 as well as other gases. The deoxygenated protoporphyrin ring has a dome-like structure because of steric hindrances and other forces from the surrounding amino acids (Figure 14-4B).
Figure 14-4.
A–B. Binding of Oxygen (O2) to Hemoglobin (Hgb) Molecule. (A) Binding of O2 by the Fe2+ atoms’ fifth (5) coordination site histidine with additional regulation by the sixth coordinate site (6) histidine. Coordination sites 1–4 are symbolically indicated in the plane of the porphyrin ring. (B) When the heme molecule’s sixth coordination site is empty, the iron molecule projects outward from the center of the protoporphyrin ring, causing the molecule to assume a domed formation. Upon O2 binding, the heme molecule assumes a planar conformation (right). [Adapted with permission from Naik P: Biochemistry, 3rd edition, Jaypee Brothers Medical Publishers (P) Ltd., 2009.]
Anemia: Anemia occurs when the quantity of RBCs is lower than normal. In severe forms of anemia, the capacity of the blood to carry adequate O2 to the peripheral tissues is compromised. Anemia can be caused by either the failure to produce enough RBCs (e.g., iron deficiency or aplastic anemia), a loss of RBCs (bleeding), an increased destruction of RBCs that cannot be compensated for by the bone marrow (e.g., sickle cell anemia or autoimmune hemolytic anemia), or by sequestration, in which RBCs are hidden away (usually in the spleen). The most common cause of anemia, and the most common nutritional deficiency in the world, is iron deficiency anemia. In this condition, there is insufficient dietary iron for erythropoiesis. The failure to meet ongoing, persistent demands of the bone marrow to create new RBCs results in vague symptoms of weakness and fatigue. Serious cases can manifest as shortness of breath and even death. |
Carbon Monoxide (CO) Poisoning: CO is a dangerous gas not only because it is undetected by our sense of smell but also because it has an affinity 200 times greater than that of O2 for Hgb’s sixth coordination position. As a result, CO will displace O2 for binding to Hgb. The displacement of O2 by CO severely decreases O2 delivery to the peripheral tissues and decreases the cell’s ability to carry out oxidative phosphorylation. The detection of CO poisoning can be difficult. Notably, a patient does not have to appear blue to be in dire need of O2; patients are just as pink when Hgb is saturated with either O2 or CO. The treatment for CO poisoning is to first remove the patient from the source of CO exposure and, in extreme cases, to put the patient in a hyperbaric O2 chamber. In a hyperbaric O2 chamber, the equilibrium between Hgb binding O2 and CO is changed and the high-pressure O2 displaces the CO molecule from the sixth coordination position of the Hgb molecule. |
Diseases Associated with Inadequate Synthesis of Hemoglobin Components
Several disease states are associated with the inadequate synthesis of any of the final Hgb components. Of the four components of Hgb (the protoporphyrin ring, α-globin, β-globin, and iron), cells must synthesize all but iron, which it absorbs from the intestinal tract. These diseases are summarized in Table 14-1.
Condition/Cause | Types | Clinical Manifestations |
---|---|---|
Porphyrias—inability to efficiently synthesize the protoporphyrin ring. | NA | These rare diseases display a spectrum of manifestations: acute abdominal pain, skin and tooth discoloration, and hemolysis [destruction of red blood cells (RBCs)]. An inciting agent such as an environmental exposure (e.g., sunlight) or drug (e.g., barbiturate) often precipitates clinical manifestations. |
Thalassemias*—defect in the amount of globin chains that are synthesized. There are four α-globin genes and two β-globin genes. | α-Thalassemia—underproduced α-globin subunit because of mutated α-globin genes. | Carrier state—A single α-globin gene is mutated. No clinical manifestations. |
α-Thalassemia trait—Two α-globin genes are mutated. There is a mild microcytic anemia (small RBCs). This anemia is usually not clinically relevant but must be distinguished from iron deficiency, discussed below. In contrast to iron deficiency, in α-thalassemia trait, blood iron studies are normal. | ||
Hemoglobin (Hgb) H—Three of four α-globin genes are mutated. There is not enough α-globin made to match the demands of the body. This is treated either with chronic RBC transfusions or by bone marrow transplantation. | ||
Hgb “Barts”—All four α-globin genes are mutated. This condition is not compatible with life, and results in hydrops fetalis and intrauterine death (α-globin is required for normal fetal development). | ||
β-Thalassemia—underproduced β-globin subunit because of mutations in the β-globin genes. | β-Thalassemia trait—One β-globin gene is mutated. Like α-thalassemia trait, β-thalassemia trait causes a mild microcytic anemia that must be distinguished from iron deficiency. | |
β-Thalassemia major and β-thalassemia intermedia—Both β-globin genes are mutated. Patients requiring chronic blood transfusions are classified as major; those who do not require are classified as intermediate. | ||
Iron deficiency anemia—inadequate iron supply to sustain erythropoiesis. | NA | Believed to affect one billion people. Symptoms include pallor, weakness, and lethargy. Severe and prolonged iron deficiency can result in neuropsychological problems. Treatment is by addition of iron to the diet via tablets. |
Oxygen Binding
Each Hgb unit is in equilibrium between two different structural states: tense (T) or relaxed (R) (Figure 14-5). Hgb in the T state has a low affinity for O2 and is more likely to surrender O2 molecules it is holding. Hgb in the “R” state has an approximately 100-fold increase in affinity for O2 as compared with the T state; R state Hgb is less likely to surrender O2 to tissues. A mutation in a globin gene or a small molecule that stabilizes the T state (or destabilizes the R state) will result in a higher proportion of O2-binding units being in the T state. An overall shift to the right in the O2 dissociation curve will occur (lower affinity for O2) (Figure 14-6). Conversely, mutations or small molecules that destabilize the T state (or stabilize the R state) will result in a higher proportion of O2-binding units being in the R state. An overall shift in the O2 dissociation curve to the left (higher affinity) will occur.
Figure 14-5.
Hemoglobin Binding of Oxygen (O2) Causes a Change in the Shape of the Hemoglobin (Hgb) Molecule. Hgb molecules exist in an equilibrium of deoxy/tense and oxy/relaxed states. Deoxy Hgb (left) has a low affinity for O2 and is associated with the tense (T) state and the release of O2 to the tissues. Binding of O2 causes an approximately 15° rotational change in the quaternary structure to form oxy Hgb (right). Oxy Hgb has a high affinity for O2 and is associated with the R state. Oxy Hgb will bind O2 more than release O2. [Reproduced with permission from Murray RA, et al.: Harper’s Illustrated Biochemistry, 28th edition, McGraw-Hill, 2009.]
Figure 14-6.
The Oxygen (O2)–Hemoglobin (Hgb) Dissociation Curve. The O2–Hgb dissociation curve depicts the saturation of Hgb with O2 as a function of the partial pressure of O2 (pO2). pO2 is a measure of the concentration of O2 in the tissues. The pO2 of metabolically active tissues is approximately 40 mmHg. At pO2 = 40 mmHg, theaffinity for O2 steeply declines and Hgb surrenders O2 to the tissues. [Adapted with permission from Kibble JD and Halsey CR: The Big Picture: Medical Physiology, 1st edition, McGraw-Hill, 2009.]
Each of the four subunits of Hgb contains one protoporphyrin ring, thereby allowing a total of four O2 molecules to bind per Hgb molecule. However, O2 binding is not a simple, linear process. The R and T states exist in equilibrium with each other and this equilibrium is what determines the overall sigmoid or “S” shape of the O2 dissociation curve (Figure 14-6). The binding of the first O2 to a single Hgb site sets in motion a series of conformational changes of the globin, polypeptide chains that increase the probability that the other three sites will adopt the “R” state (the high affinity state). Binding of subsequent O2 molecules also increases the binding of the other sites (although much less than the binding of the first O2). In addition, more relative R state than T state shifts the curve to the left. Conversely, more T than R will shift the curve to the right.
The role of Hgb is to transport O2 from higher partial pressure of O2 (pO2) environments (such as lungs) to lower pO2 environments (such as tissues). Because the ligand affinity of Hgb is increased by successive binding, O2 is a positive allosteric effector (Chapter 5). The S shape of the O2–Hgb dissociation curve depicts a positive cooperativity relationship between the percent saturation of the Hgb molecule and pO2 (Figure 14-6). The positive cooperativity of O2 binding is an essential characteristic of Hgb that makes it both an efficient O2 carrying and delivery molecule. This characteristic also allows more O2 delivery to tissues with high metabolic demands (low pO2). Correspondingly, tissues that need less O2 because of fewer metabolic demands have higher pO2 and extract, appropriately, less O2.
An important concept in understanding Hgb function is that Hgb must be able to carry out two seemingly opposing functions: (1) to become fully saturated with O2 in the lungs and (2) to be able to efficiently unload O2 in the tissues. That is, O2 binding does not guarantee O2 delivery. In fact, if Hgb binds O2 too tightly, tissues would not be able to extract O2 from the Hgb molecule, and functionally it would be equivalent to there being no O2 at all. It is the release of O2 from Hgb that drives tissue oxygenation. Negative allosteric regulators such as increased temperature, increased CO2, and decreased pH (increased H+ concentration) will cause the curve to shift to the right and help Hgb unload O2 in the tissue (Figure 14-7).
Figure 14-7.
Effects of Temperature and pH on Hemoglobin (Hgb) Binding of Oxygen (O2). (A) Increasing temperature (e.g., 10, 20, 38, and 43°C) causes reduced binding of O2 by Hgb, reflecting reduced affinity for O2 (T state > R state). In this higher temperature environment, Hgb will more readily release O2 as noted by the right shift of the curves. (B) Increasing pH (e.g., 7.2, 7.4, and 7.6) causes increased binding of O2 by Hgb. As the curve shifts left, Hgb has an increased affinity for O2 (R state > T state). [Adapted with permission from Kibble JD and Halsey CR: The Big Picture: Medical Physiology, 1st edition, McGraw-Hill, 2009.]
2,3-bisphosphoglycerate (2,3-BPG)
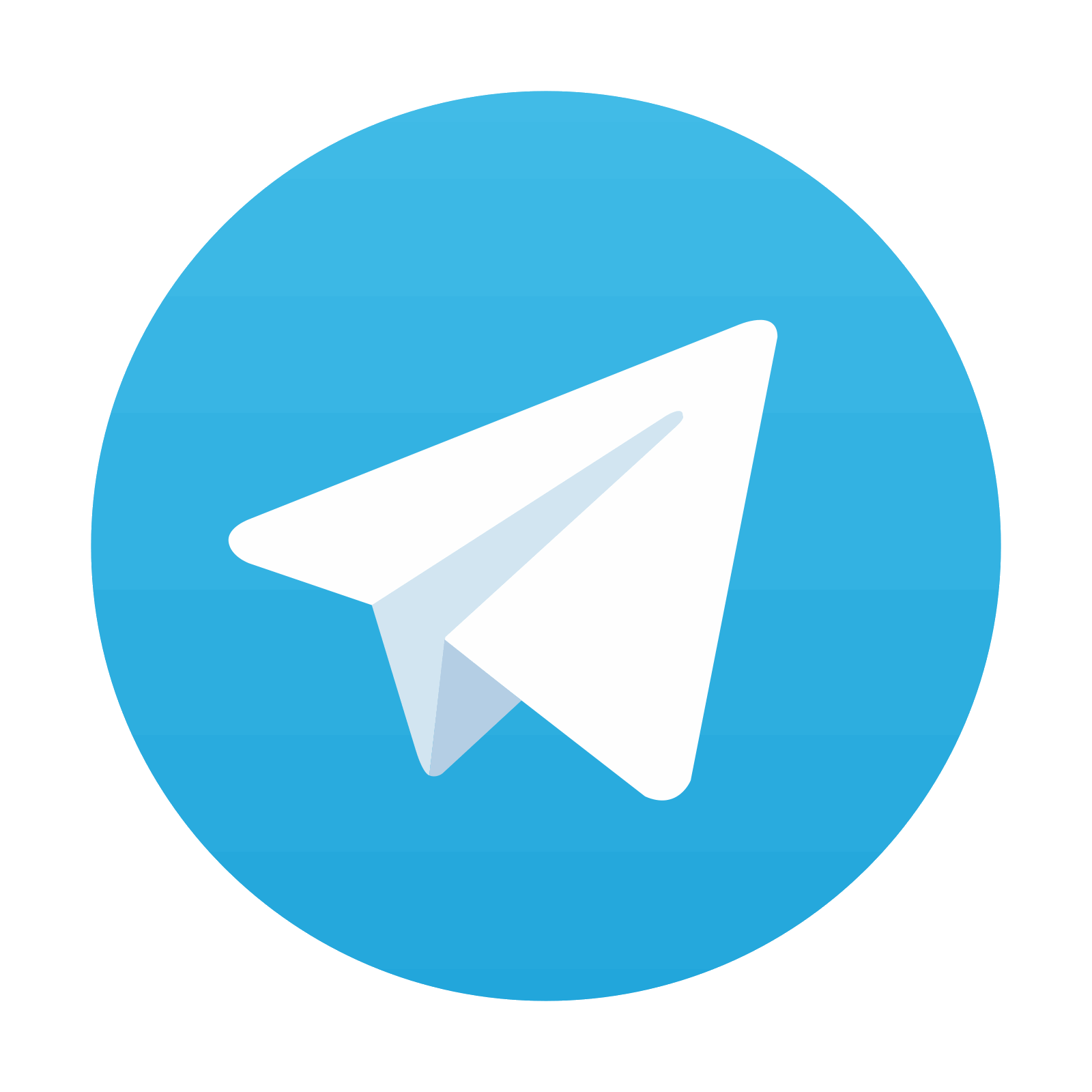
Stay updated, free articles. Join our Telegram channel
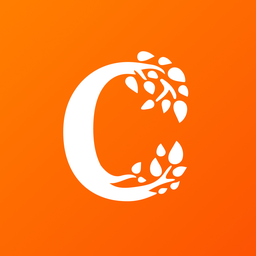
Full access? Get Clinical Tree
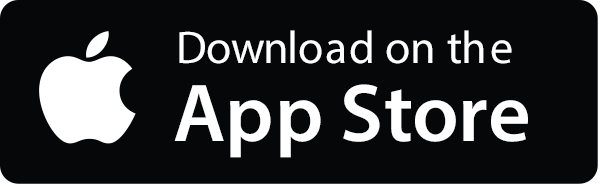
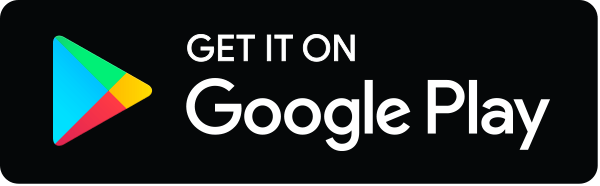
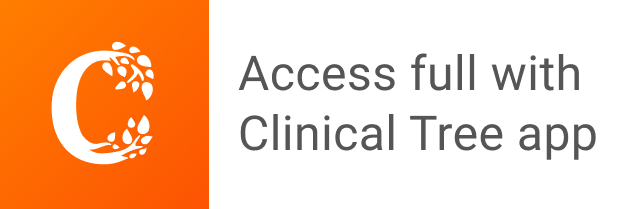