Overview
Movement and its regulation, on a microscopic and/or macroscopic scale, are essential for human life. Muscle, composed of actin, myosin, and a variety of structural and regulatory proteins, is one of the main cell/tissue types involved in this movement. Skeletal, cardiac, and smooth muscles offer a coordinated and regulated means to move the human body from place to place; interact with its surroundings; keep nutrients flowing to and waste products flowing from various cells; or move nutrients, blood, lymph, and other molecules. Specialized proteins and the means to provide energy for these processes have evolved for particular tissues and functions.
Microtubules with tubulin, dynein, and kinesin molecules and associated structures such as cilia, flagella, centrioles, basal bodies, centromeres, and mitotic spindles provide another important mechanism for a variety of cell movements and internal cell functions that affect all types of cells and allow the division of cells. Intermediate filaments (IFs) also serve several essential roles of cell motility. Nonmuscle cells utilize all of these mechanisms—actin/myosin, microtubule/dynein/kinesin, and IFs—to achieve a wide array of functions throughout the human body.
The Basic Components of Muscle
Muscle is an organ that specializes in transforming chemical energy into mechanical work or movement. The muscular system comprises all the individual anatomic muscles. Muscle tissue is derived from the mesodermal layer of embryologic germ cells and is divided into three main types: skeletal muscle, cardiac muscle, and smooth muscle (Figure 12-1). The structure of these different types of muscles is similar but the architecture and regulation are often very different, making their functions unique. Nonmuscle cells are a fourth cell type that utilizes many of the same proteins and processes for motility.
Figure 12-1.
A–C. The Three Types of Muscle. Light micrographs of each type, accompanied by labeled drawings. A. Skeletal muscle is composed of large, elongated, multinucleated fibers that show strong, quick, voluntary contractions. B. Cardiac muscle is composed of irregular branched cells bound together longitudinally by intercalated disks and shows strong, involuntary contractions. C. Smooth muscle is composed of grouped, spindle-shaped cells with weak, involuntary contractions. The density of intercellular packing seen reflects the small amount of extracellular connective tissue present. [Reproduced with permission from Mescher AL: Junqueira’s Basic Histology Text and Atlas, 12th edition, McGraw-Hill, 2010.]
There are two major proteins involved in contraction in all types of muscle: actin and myosin. These proteins convert chemical energy into mechanical work through an interaction with adenosine triphosphate (ATP). The triggering and control of this process are different for each muscle type. Skeletal, cardiac, and smooth muscles have a repeating unit called the sarcomere, containing the muscle fibers composed of actin and myosin and accessory proteins (see below). Surrounding these muscle fibers is the equivalent of a plasma membrane called the sarcolemma. Within the sarcolemma is the sarcoplasm containing mitochondria and a sarcoplasmic reticulum (SR), the equivalent of a smooth endoplasmic reticulum (SER). Like the SER in other cell types, the SR contains calcium ions (Ca2+) essential for the initiation of muscle activation. More specifically, specialized invaginations of the SR, called transverse tubules (T-tubules), interdigitate between the muscle fibers to provide efficient delivery of calcium.
The term actin describes both the globular, single amino acid (monomer) chain (G-actin) and the thin filament (F-actin) structure formed from two parallel strands of multiple G-actin molecules, which wind to form a double helix structure (Figure 12-2A). Three major classes of actin exist, including α-actin (found in skeletal, cardiac, and smooth muscles) and β- and γ-actins (found in nonmuscle cells). These F-actin filaments provide structure as part of the cell’s cytoskeleton. The cytoskeleton is also directly linked to the plasma membrane via integral membrane proteins and intracellular junctions (Chapter 8) and, as such, is important in many signaling functions.
Figure 12-2.
A–C. Molecules Composing Thin and Thick Filaments. The contractile proteins are the thin and thick myofilaments within myofibrils. A. Each thin filament is composed of F-actin, tropomyosin, and troponin complexes. B. Each thick filament consists of many myosin heavy chain molecules bundled together along their rod-like tails, with their heads exposed and directed toward neighboring thin filaments. C. Besides interacting with the neighboring thin filaments, thick myofilament bundles are held in place by less well-characterized, myosin-binding proteins within the M-line. [Reproduced with permission from Mescher AL: Junqueira’s Basic Histology Text and Atlas, 12th edition, McGraw-Hill, 2010.]
Actin thin filaments interact with myosin thick filaments (Figure 12-2C and see below) and are integral in a multitude of cell motility functions, including muscle contraction, the movement of intracellular vesicles, cell division and cytokinesis, immune response, phagocytosis, wound healing, and others. Actin is also required in the nucleus for RNA polymerase I, II, and III complex formation and function, export of RNA and proteins from the nucleus, and some aspects of chromatin remodeling.
Tropomyosin is a regulatory protein that binds to F-actin thin filaments and reversibly blocks myosin head binding sites (Figure 12-2A–C). In noncontracting muscle, troponin T and troponin I molecules (part of a troponin complex, Figure 12-2A) hold tropomyosin over the myosin-binding site. Upon exposure to Ca2+ released from the SR, troponin C, the third protein of the troponin complex, creates a conformational change of the tropomyosin–troponin complex exposing the site. When calcium concentrations fall, the change reverses and the block is again present. Smooth muscle and nonmuscle cells do not contain troponin and rely on other regulatory processes to control actin–myosin contraction/force generation. Troponin C contains a specific amino acid sequence named an EF hand. In this tertiary structure motif (Chapter 1), an α-helix (termed E for its sequence in a series of α-helices) is joined to an F α-helix by a loop of approximately 12 amino acids. The helix–loop–helix forms a structure reminiscent of an extended forefinger (E helix) and thumb (F helix) with the curve between representing the loop. Among the 12 amino acids of the loop are positions, 1, 3, 5, 7, 9, and 12, which bind the Ca2+. Amino acid 12 is always glutamate or aspartate whose R-group provides two partially negatively charged oxygen molecules (Chapter 1) for binding the positively charged calcium. The small size of a highly conserved glycine amino acid at residue 6 provides space for this structure to form. The other amino acids are mainly hydrophobic that stabilize the E and F helices and, therefore, the helix–loop–helix structure.
The human genome holds more than 40 different types of myosin genes. These different genes produce variations of myosin shapes, which in turn affect the speed at which the filaments move. To date, there are 12 classes of myosin defined in the human genome. All myosin molecules have three domains, including a head, neck, and tail portion of the amino acid sequence (Figure 12-3A). The myosin head binds actin thin filaments and uses hydrolysis of ATP to adenosine diphosphate (ADP) to generate force. The tail domain varies depending on the type of myosin but, in general, can bind to transport vesicles or combine with other myosin molecules. Some myosin tail domains can also regulate the proteins— activity by folding over and effectively blocking the myosin head domain. The neck domain serves as a link or lever arm for the transfer of this force between the head and tail. The varying types of myosin proteins produce different speeds of movement, depending on the length of the neck region. Four myosin light chains: two essential light chains, and two regulatory light chains are also bound to the neck regions (Figure 12-3A). The function of these light chains is discussed below.
Figure 12-3.
A–C. Myosin I and II. A. Basic Myosin Molecule. The heavy and light chains (essential–red, regulatory–dark blue) form the basic structure of all myosin molecules, including the long tail composed of two coiled α-helices and the globular head region. B. Myosin I. Vesicles for transport are attached by the myosin I tail section. The single head of myosin I moves down the actin filament in a similar manner to other myosin heads. Myosin I molecules can also link between F-actin filaments, resulting in limited motility similar to myosin II molecules. [Adapted with permission from Naik P: Biochemistry, 3rd edition, Jaypee Brothers Medical Publishers (P) Ltd., 2009.] C. Myosin II. The tail section of the two heavy chains of myosin II forms a coiled-coil arrangement. This structure can interact with several other myosin II tails to form thick filaments. The two head regions of each myosin molecule contain an actin-binding site (red circle) and an ATP-binding site (blue line). Coordinated movement of the myosin heads produces force and, therefore, movement when interacting with actin filaments. See text for further details. [Adapted with permission from Mescher AL: Junqueira’s Basic Histology Text and Atlas, 12th edition, McGraw-Hill, 2010.]
Myosin I is the simplest form consisting of a basic myosin head, neck, and a tail of varying lengths (Figure 12-3A–B). The basic function of myosin I is most often described as vesicle transport, although other functions are emerging. Myosin II is composed of two identical amino acid chains, termed the “heavy chains” (Figure 12-3A). The tail section of each chain coils around the other, making a dimer myosin filament with two heads. Multiple myosin II dimers bind at the tail domain, forming a structure called a thick filament (Figures 12-2B–C and 12-3C). Additional myosin subtypes have structures similar to either myosin I or myosin II and are responsible for diverse types of cell motility. These functions include intracellular transport (both to and from the nucleus to the cell periphery), maintenance and movement of vesicles and organelles in subregions of the cytoplasm, and, possibly, the perception of light in the retina and sound waves in the inner ear.
Each myosin head contains two essential myosin light chains and two regulatory myosin light chains bound to the neck region (Figure 12-3A). Different forms of myosin light chain are found on different myosin types and influence their activity and regulation. Myosin light chains serve an important regulatory role for myosin activity in smooth and nonmuscle cell contraction where the tropomyosin–troponin mechanism is absent. In skeletal muscle, the myosin light chains influence the speed of contraction but are not essential for myosin activity. In cardiac muscle, myosin light chains affect myosin head ATPase and are believed to have some role in the development of heart failure. Activity of myosin in smooth/nonmuscle cells relies on phosphorylation of serine 19 on the regulatory chains by myosin light chain kinase (MLCK). MLCK activity is regulated by Ca2+ that binds to the regulatory protein calmodulin, also by an EF hand-binding motif (see above). Calmodulin subsequently activates MLCK. When calcium concentrations fall, myosin light chain phosphatase (MLCP) removes the phosphate group and inactivates the regulatory light chains and, therefore, myosin. Calcium also inhibits MLCP by the actions of another serine/threonine enzyme called Rho kinase; other signaling pathways and enzymes have also been implicated in myosin light chain phosphorylation and dephosphorylation. One enzyme, sphingosine-1-phosphatase, may play an important role in modulating contraction and relaxation of blood vessels and, therefore, maintenance of blood flow in response to changes in blood pressure.
Actin-binding proteins (ABPs) are a varied group of proteins that regulate actin filament formation and length as well as actin–myosin interactions. Although more prominent in nonmuscle cell and smooth muscle contraction, some, including tropomyosin and the troponins (see above), are found in skeletal and cardiac muscles. One such ABP, α-actinin binds actin thin filaments to skeletal muscle Z-lines and smooth muscle dense bodies (Figures 12-4 and 12-8A). α-Actinin is also believed to bind to actin filament bundles and separates each thin filament by approximately 35 nm. This separation allows myosin thick filaments the proper spacing for optimal contraction. Additional ABPs cross-link thin filaments (filamin), sever thin filaments (gelsolin, tropomodulin, and villin) and cap them to restrict their length (capZ), regulate actin–tropomyosin activity in smooth muscle cells where troponins are absent (caldesmon), regulate myosin ATPase activity (calponin), and connect microfilaments to the cell membrane (dystrophin, vinculin, and integrins). Many other ABPs also exist and perform other functions in a variety of cell types.
Figure 12-4.
Excitation–Contraction Coupling. Actin and myosin form sarcomere structures in skeletal muscle as shown. The influx of calcium ions (Ca2+) allows myosin heads from thick filaments to bind to the actin filaments. Ca2+ also activates myosin head cross-bridge cycling, leading to force generation and, ultimately, shortening of the sarcomere. Shortening of multiple sarcomeres leads to muscle contraction. The process is similar in cardiac and smooth muscle contraction, although structural organization and regulation differ. ADP, adenosine diphosphate; ATP, adenosine triphosphate. [Reproduced with permission from Kibble JD and Halsey CR: The Big Picture: Medical Physiology, 1st edition, McGraw-Hill, 2009.]
Excitation–Contraction Coupling
Muscle achieves its major function of generating movement by contraction through a series of binding events and enzymatic reactions. This process is called excitation–contraction coupling (Figure 12-4). Certain parts of this mechanism are conserved in all types of actin–myosin contraction/motility, whereas alternative forms of initiation and/or regulation are found in particular muscle types. Many of the components are similar in nonmuscle cells, but many differences exist because of the variations in organization and additional proteins and their functions. Nonmuscle cell contraction will be discussed separately below.
Two basic processes must happen in all muscle types for contraction to occur: (1) binding of myosin heads to the actin thin filament and (2) stimulation of myosin to generate force. Both of these processes rely, at least in part, on Ca2+. In all muscle types, a signal from a nerve or pacemaker cells causes an initial increase in Ca2+ concentrations. This relatively small influx of Ca2+ leads to a larger release from the SR. This concept of a small calcium signal leading to higher calcium concentrations and contraction is termed calcium-induced calcium release (CICR). The release from the SR varies depending on the type of muscle and is discussed below. T-tubules allow delivery of this calcium to all areas of a muscle unit to permit synchronous contraction. The released calcium interacts with an ABP—troponin/tropomyosin in skeletal and cardiac muscle and caldesmon in smooth muscle—to allow myosin head binding to actin thin filaments.
Myosin force generation is the second basic function required for contraction. In all muscle types, ATP binds to the myosin molecule head. An ATPase on the myosin head cleaves ATP into ADP and a phosphate molecule (PO43−), producing a charged form of the myosin protein that binds to the now open F-actin filaments at an angle. Release of the phosphate molecule from the myosin head causes it to swivel to a more acute angle, resulting in a ratcheting movement between it and actin. This ratcheting movement slides the thick and thin filaments past each other, converting the chemical energy of the ATP into the mechanical energy moving the filaments. Release of the ADP molecule and binding of a new ATP molecule breaks the bond between myosin head and F-actin thin filament. As this process repeats many times, the overall length of the sarcomere shortens. Calcium also plays a prominent role in smooth muscle myosin activity via activation of regulatory myosin light chains (see above).
The cycle continues as long as the concentration of calcium in the sarcoplasm of the cell remains elevated. At the end of this process, the calcium entry into the cells slows and begins to be collected again by the SR by a sarco/endoplasmic reticulum Ca2+-ATPase pump, also powered by ATP. The pump can produce an approximately 10,000-fold higher concentration inside the SR versus the sarcoplasm. An additional protein calsequestrin also binds Ca2+ inside the SR to reduce the effective, free Ca2+ concentration that the pump must work against. Calsequestrin can bind over 40 Ca2+ per protein molecule not by an EF hand structure but, instead, by using charged amino acids residues and changes of secondary structure to α-helices. Once the calcium concentration lowers, calcium is released from troponin C, the previous conformational change is reversed and troponin T and I again block the binding of myosin to the actin binding site. As the cycle ends, a new molecule of ATP then binds to the myosin head, which displaces the ADP and the initial sarcomere length is restored.
Skeletal Muscle
Skeletal muscle comprises the bulk of the muscle mass found in our bodies (Figure 12-5). The average adult male is made up of approximately 42% skeletal muscle and an average adult female consists of 36% skeletal muscle. Each muscle has an origin and insertion on the skeleton, with the individual muscle fibers running parallel or oblique to the long axis of the muscle. Skeletal muscles are capable of producing a forceful contraction and moving the elements of the muscle over a relatively large distance. With the shortening of length during the contraction, this muscle will often change in diameter, like the swell produced when contracting the biceps muscle. In skeletal muscle, the F-actin thin filaments are bound end to end at a structure called the Z-line (Figure 12-4). The repeated Z-lines and alternating thin and thick filaments give the muscle the striated appearance seen on light microscopy.
Figure 12-5.
Skeletal Muscle. Skeletal muscle is highly organized. At the molecular level, repetitive sarcomeres (see text above) form a myofibril. Multiple myofibrils form muscle cells, columns, and, ultimately, muscle. [Reproduced with permission from Kibble JD and Halsey CR: The Big Picture: Medical Physiology, 1st edition, McGraw-Hill, 2009.]
Centronuclear/Myotubular Myopathies: Centronuclear/Myotubular myopathies (CNMs) are a rare series of inherited disease states in which skeletal muscle nuclei are located in the center of the muscle cells instead of at the peripheral edges. The exact causes have not been found but errors in the development of embryological muscle cells appear to be common. A suspect enzyme called myotubularin, which has dephosphorylating activity, may be part of the X-linked form, properly termed myotubular myopathy. X-linked CNM presents at birth with severe loss of muscle tone (hypotonia), affects muscles required for breathing, and often results in death before a cause can be determined. Patients also often have an associated narrow and long head with palate, finger, and chest structural abnormalities. Autosomal dominant and recessive forms also exist, properly termed centronuclear myopathies. The autosomal dominant version may be because of mutations affecting the protein dynamin, a guanosine triphosphate-dependent transport protein. Symptoms of CNM may never present (e.g., autosomal recessive form) or may include variable ranges of increasing muscular weakness, which often presents in the teens or 20s. There is no cure for CNM diseases and treatment is only supportive. |
Growth, regeneration, and activity of skeletal muscle is under the control of several signaling pathways, which activate specific gene transcription/translation, leading to the production of muscle proteins as well as regulatory proteins, including those involved in energy production for muscle contraction. Some are dependent on motor neuron activity and/or contraction [e.g., more glucose transporter type 4 (GLUT4) receptors are brought to the surface in highly contracting muscles to increase glucose uptake] and, therefore, are directly influenced by specific muscle activity.
Skeletal muscle is under voluntary control and the contraction is driven by motor neurons in the spinal cord and the neurotransmitter acetylcholine
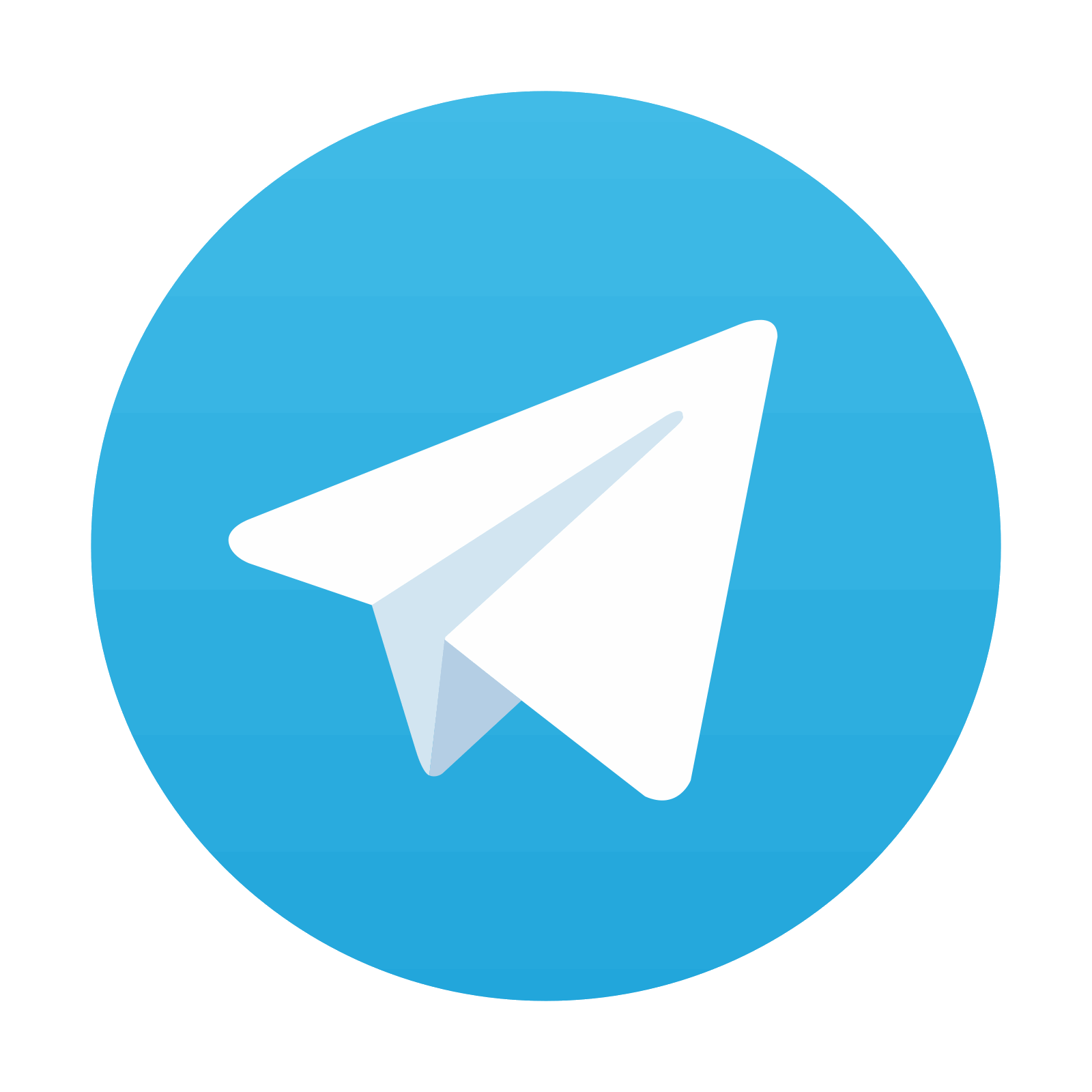
Stay updated, free articles. Join our Telegram channel
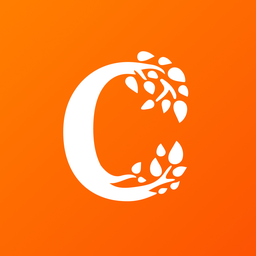
Full access? Get Clinical Tree
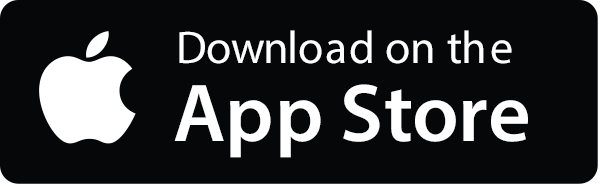
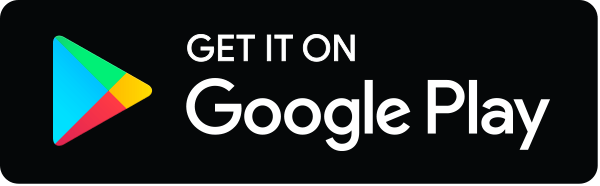
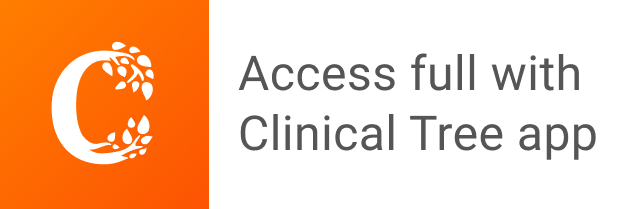