Overview
The integration of metabolism is a story of supply and demand. Food is ingested to supply energy but must be converted to the carbohydrate, lipid, and amino acid forms the body can use, primarily glucose and fatty acids. Individual cells then convert the fuels to usable energy, adenosine triphosphate (ATP) and nicotinamide adenine dinucleotide (NADH). The body demands energy to function but individual organs and tissues require particular sources of energy under varying conditions.
To convert consumed food into the needed energy, the body uses a variety of organs, each with unique metabolic profiles, to integrate and regulate the use and storage of energy. Specific regulatory points of biochemical pathways provide immediate control of the usage, conversion, or storage of food energy. Various hormones can also regulate these biochemical pathways to provide longer term control of food conversion and energy usage. Essential to both of these processes is the maintenance of glucose homeostasis. Finally, vitamins and minerals serve important functions as cofactors in many of these metabolic reactions. Their deficiency or excess can lead to numerous disease states.
Metabolic Roles of Major Biochemical Molecules
The first consideration is the major sources of energy that can be used by the body, the nutrients required for their metabolism, and the biochemical pathways that integrate them.
Amino acids (Chapter 1, Figure 10-1) provide several major biochemical functions, including serving as (1) the building blocks of proteins; (2) the precursors of hormones, neurotransmitters, and other important signaling molecules (such as nitrous oxide); and (3) contributors to the purine and pyrimidine components of nucleic acids, co-enzymes [NADH and flavin adenine dinucleotide (FADH2)], and other fundamental biological molecules. Additionally, excess amino acids can enter the citric acid cycle and can be used to generate or store biological energy (Chapter 5). Furthermore, the metabolism of some amino acids can be funneled into glucose synthesis (gluconeogenesis) during food deprivation.
Carbohydrates (Chapter 2, Figure 10-2) perform a fundamental role as the primary energy-production source for the human body. Glycolysis and the subsequent metabolic pathways form the primary energy molecules ATP, NADH, and FADH2 via the oxidation of glucose and other carbohydrates (Chapter 6). Storage of carbohydrates as glycogen offers a readily available source of energy when dietary carbohydrate intake is low (Chapter 2). Carbohydrates are also important in the synthesis of nicotinamide adenine dinucleotide phosphate (NADPH) (Chapter 6) and nucleic acids (Chapter 4).
Lipids (Chapter 3, Figure 10-3) are nonpolar biomolecules. In most tissues, they serve a primary structural role as the components of biological membranes, creating a lipid bilayer via their hydrophobic and hydrophilic entities (Chapters 7 and 8). Their roles in membranes as well as in pathological processes such as atherosclerosis (Chapter 16) have raised the awareness of saturated, mono-unsaturated, and poly-unsaturated forms with regard to their role in diet. However, in adipose tissue, triglycerides are the major storage form of biological energy and their oxidation yields more energy per carbon than carbohydrates (Chapter 7). Lipolysis of triglycerides mobilizes fatty acids that generate energy through β-oxidation and produces the substrates necessary for ketone body (acetoacetate and β-hydroxybutyrate) synthesis, an essential fuel source during prolonged starvation. Oxidation of both fatty acids and ketone bodies spares glucose by preventing its oxidation. The consumption of dietary cholesterol and fats has a large impact on lipid metabolism through the generation of plasma lipoproteins [chylomicrons and low-density lipoprotein (LDL) via very-low-density lipoprotein (VLDL)]. The resultant elevation of harmful lipids/lipoproteins (dyslipidemia) has negative metabolic consequences that directly impact health and disease throughout all socioeconomic classes of modern society.
Figure 10-3.
Transport and Fate of Major Lipid Substrates and Metabolites. FFA, free fatty acids; LPL, lipoprotein lipase; MG, monoacylglycerol; TG, triacylglycerol; and VLDL, very-low-density lipoprotein. [Reproduced with permission from Murray RA, et al.: Harper’s Illustrated Biochemistry, 28th edition, McGraw-Hill, 2009.]
Vitamins, both lipid and nonlipid derived, serve important roles as cofactors in metabolic pathways and reactions (see end of this chapter). Several diseases, including scurvy, rickets, and Wernicke–Korsakoff syndrome, result directly from deficiencies of vitamins or, as in pernicious anemia, from the body’s inability to properly absorb them. Minerals, including sodium, potassium, chloride, calcium, phosphate, iron, and others, play major roles in the regulation of metabolic enzymes involved in digestion, in the use and/or storage of food metabolites, and in the elimination of waste products.
Even more important is the integration of metabolism of these molecules in the human body and how regulation can be maintained by interrelationships between their anabolic and catabolic metabolism. In this regard, the body’s ability to sense energy levels, respond to hormone signaling, and upregulate and downregulate particular metabolic pathways is paramount for the body to maintain the proper and controlled level of metabolic function and for the myriad of structural and functional processes to occur, which allow life.
Integration and Regulation of Metabolism
ATP, associated with magnesium (Mg2+) for stability, is the primary form of biological energy utilized by the human body (Figure 10-4).
As such, the catabolic oxidation of carbohydrates (glycolysis, citric acid cycle, and oxidative phosphorylation), fatty acids/lipids/ketone bodies (fatty acid degradation), and amino acids all lead eventually to the production of ATP. In contrast, anabolic metabolic processes (gluconeogenesis, glycogen synthesis, lipid synthesis, triglyceride synthesis, and amino acid synthesis) consume ATP, NADH, and/or NADPH to store energy (glucose), to store energy, or to build essential biomolecules. Coupled to all of these processes is the need to eliminate waste products, including CO2 (exhalation, acid–base balance), reactive and/or free-radical species (antioxidants), and urea (urea cycle). These concepts are summarized in Figure 10-5.
These metabolic pathways are intimately linked at several points in biochemical pathways, but are also separated into distinct compartments and/or organelles (e.g., cytoplasm versus mitochondria versus nucleus, etc.) to allow the necessary regulation and control. Additionally, each organ has unique metabolic needs and functions as summarized in Figure 10-6. These functions and needs must be coordinated in a variety of organs to maintain a constant supply of energy while preserving some energy for the future. The body accomplishes this goal by using the nervous system and hormonal signals to differentially stimulate and inhibit biochemical pathways within various organs in response to supply and demand. The main signals used to regulate metabolism are insulin, glucagon, catecholamines, glucocorticoids, and growth hormone (in children).
The remainder of this chapter will focus on the metabolism in three major tissues, the liver, adipose tissue, and skeletal muscle (Figure 10-6). The liver actively provides the quick fuel (glucose) your body needs, whereas adipose tissue provides long-term energy storage. Finally, skeletal muscle and the rest of your body constantly demand this energy. For example, the brain consumes approximately 90 g of glucose in a day, 20% of the average diet.
The supply and demand of energy must be continuously provided via dietary intake or breakdown of stores to balance with the energy requirements of respiration, transport, motility, and synthesis of cells and tissues (Figure 10-7). Overall, the average adult uses approximately 24 kcal of energy per kilogram of body mass to insure proper health and to maintain proper weight.
Several key biomolecules (glucose-6-phosphate or G6-P, pyruvate, and acetyl coenzyme A or acetyl-CoA) link the biochemical pathways for carbohydrates, lipids, and amino acids/proteins and the pathways they funnel into are tightly regulated and tissue specific (Figure 10-8). G6-P, pyruvate, and acetyl-CoA link the anabolic and catabolic pathways of carbohydrate metabolism to maintain a constant supply of energy to maintain homeostasis under constantly changing conditions. The particular pathways and regulation also depend on the specific functions and needs of each tissue type.
Figure 10-8.
Summary of Important Control Points of Metabolism. The three important intermediaries, glucose-6-phosphate, pyruvate, and acetyl-CoA are indicated. Metabolic pathways of importance are indicated in red. See text for full discussion. ATP, adenosine triphosphate; CoA, coenzyme A; NADPH, nicotinamide adenine dinucleotide phosphate. [Adapted with permission from Naik P: Biochemistry, 3rd edition, Jaypee Brothers Medical Publishers (P) Ltd., 2009.]
Metabolic regulation at this first branch point, G6-P, is clearly illustrated in the liver (Figure 10-8). After the ingestion of carbohydrates, glucose taken up by the liver is converted to G6-P by glucokinase. This phosphorylation uses one ATP molecule and traps the glucose within liver cells (hepatocytes). Subsequently, G6-P is metabolized via one of the following three pathways: (a) glycogenesis—the storage of carbohydrates as glycogen, (b) glycolysis—the production of ATP, or (c) the pentose phosphate pathway—the production of NADPH and/or five-carbon (pentose) sugars (Chapter 6, Figure 10-8). The pathway chosen depends upon the activation state of key enzymes (glycogen synthase and phosphofructokinase-1), substrate availability (G6-P, ATP, and NADP+), and allosteric effectors [ATP, adenosine monophosphate (AMP), fructose 2,6-bisphosphate (F2,6BP), hydrogen ions (H+), and citrate]. The key enzymes in glycogenesis and glycolysis are predominantly regulated by hormone-stimulated, covalent modification (phosphorylation), whereas the allosteric effectors fine-tune the flow of carbons through these pathways. In contrast, the pentose phosphate pathway is primarily regulated by the availability of G6-P and NADP+ (Chapter 6).
In the well-fed state, when ATP and citrate concentrations are high, phosphofructokinase-1 is allosterically inhibited, slowing down the committed step of glycolysis (the production of fructose 1,6-bisphosphate) leading to increased concentrations of G6-P. The increased concentration of G6-P can stimulate carbohydrate storage in two ways. First, G6-P is a positive allosteric effector of glycogen synthase, leading to the formation of glycogen. Second, G6-P indirectly inhibits glycogen phosphorylase thereby inhibiting glycogenolysis (glycogen degradation). Alternatively, when the ratio of NADP+ to NADPH is high, G6-P can be shuttled into the pentose phosphate pathway to generate NADPH (reductive energy). This reducing power is used to synthesize a variety of biomolecules such as, fatty acids, cholesterol, nucleotides and other cofactors as needed. Under conditions where the ratio of NADP+ to NADPH is low, the pentose pathway will not operate regardless of the concentration of G6-P.
The production of glycogen in the liver is further controlled by the hormones, insulin and glucagon (see below), and the resulting phosphorylation or dephosphorylation of glycogen synthase. Degradation of glycogen is decreased concomitantly by counterregulatory dephosphorylation or phosphorylation of glycogen phosphorylase. Eating breakfast, after an overnight fast, stimulates glycogen synthesis (and inhibits glycogen breakdown) in preparation for the next period of fasting. This replenishment is controlled by the favorable high ratio of insulin to glucagon, leading to activation of glycogen synthase activity and decreased glycogen phosphorylase activity. Under these conditions, the demand for de novo synthesis of lipid will rise after the glycogen is replaced, using carbons from excess dietary carbohydrate (to synthesize fatty acids). Additionally, if cholesterol biosynthesis is active, excess acetyl Co A from fatty acid catabolism can be synthesized into cholesterol. Once lipid biosynthesis commences, the utilization of NADPH increases the NADP+/NADPH ratio favoring flux through the pentose phosphate pathway.
After a meal, the key regulator that restarts glycolysis in liver is F2,6BP. F2,6BP concentration is controlled by a bifunctional enzyme that includes both kinase and phosphatase active sites. Under conditions of a high ratio of insulin to glucagon, the bifunctional enzyme (phosphofructokinase-2/fructose 2,6-bisphosphatase) is dephosphorylated, leading to stimulation of phosphofructokinase-2. The resulting F2,6BP formed allosterically activates phosphofructokinase-1 and hence increases glycolysis while simultaneously inhibiting fructose 1,6 bisphosphatase, therefore shutting down gluconeogenesis. Following food deprivation, these events are reversed with a high ratio of glucagon to insulin, favoring phosphorylation of the bifunctional enzyme stimulating the fructose 2,6-bisphosphatase activity and leading to decreased phosphofructokinase-1 activity.
The second major branch point in the integration of metabolism is at pyruvate (Chapter 6, Figure 10-8). Pyruvate can be converted into four different substrates: lactate, alanine, oxaloacetate, and acetyl-CoA, depending upon the energy needs of a cell. Therefore, it is an important integration point where carbons are shuttled between energy storage, energy generation, and/or biosynthetic reactions. In the liver, pyruvate can undergo oxidative decarboxylation to enter the citric acid cycle and ultimately generate ATP when energy levels are low. Specifically, low energy levels inhibit the activity of an important regulatory enzyme, pyruvate dehydrogenase kinase. This inhibition prevents phosphorylation of the pyruvate dehydrogenase complex to an inactive state. Furthermore, this kinase is inhibited by NAD+, pyruvate, and sulfhydryl form of CoA (non-acetylated), substrates of pyruvate dehydrogenase. Therefore, when substrates are plentiful, pyruvate is oxidatively decarboxylated to acetyl-CoA. In the liver, pyruvate is also the point where lactate and alanine (see below) can be actively funneled into either the citric acid cycle or gluconeogenesis via pyruvate carboxylation to oxaloacetic acid (Chapter 6) when liver glycogen or blood glucose levels are low. During starvation, gluconeogenesis can produce up to 160 g of glucose in a day, half of this from amino acids. Half of this glucose will be used by the brain. As blood glucose levels stabilize and gluconeogenesis is no longer required, oxaloacetate can re-enter the glycolytic pathway at phosphoenolpyruvate or shuttle back into the mitochondria, as malate, to be used in the citric acid cycle. If energy is abundant, high NADH and acetyl-CoA concentrations activate pyruvate dehydrogenase kinase and also serve as allosteric inhibitors of enzymatic activities within the PDH complex. This effectively turns off the pyruvate dehydrogenase complex by phosphorylation and allosteric control and shuts down the citric acid cycle. High ATP and acetyl-CoA concentrations also stimulate pyruvate carboxylase, the first step of gluconeogenesis (hormone regulation of gluconeogenesis is even more important; see below).
Skeletal muscle illustrates another important way that pyruvate can be metabolized (Figure 10-9). If oxygen levels are low and anaerobic respiration becomes important (such as during a quick sprint), pyruvate can be converted to lactate by lactate dehydrogenase with an oxidation of one NADH to NAD+, the latter being essential for sustaining glycolysis. In this scenario, ATP is solely derived from anaerobic glycolysis. Lactate can subsequently be converted back to glucose for energy production via the Cori cycle (in the liver); when lactate concentrations get too high, feedback inhibition blocks further conversion of pyruvate to lactate. High lactate concentrations also create the sensation of “burning” in muscles, which serves as a signal to the body to limit further use of these muscles. Furthermore, pyruvate can also be converted in muscle tissue to the amino acid alanine via the alanine transaminase reaction. In a manner analogous to the Cori cycle, the alanine cycle then converts this alanine back to pyruvate in the liver where it is used to produce new glucose via gluconeogenesis as a source of energy for anaerobic glycolysis in muscle. Once oxygen levels are restored in skeletal muscle, production of ATP via citric acid cycle/oxidative phosphorylation resumes.
Figure 10-9.
The Lactic Acid (Cori) and Glucose–Alanine Cycles. Carbons from glucose metabolism in muscle are recycled to the liver either as lactate or alanine for reconversion to glucose. Hence, when these cycles operate glucose carbons are spared. [Adapted with permission from Murray RA, et al.: Harper’s Illustrated Biochemistry, 28th edition, McGraw-Hill, 2009.]
Acetyl-CoA is the third branch point of primary metabolic control, and coordinates carbohydrate, ketone, and fat/lipid pathways (Chapter 6, Figures 10-8 and 10-10). Acetyl-CoA is a substrate for the citric acid cycle and can be oxidized to generate energy. However, when energy levels are high (high NADH/NAD+ ratio), NADH inhibits the citric acid cycle at the isocitrate dehydrogenase and α-ketoglutarate dehydrogenase steps. Accumulation of FADH2 also occurs, leading to an increase in succinyl-CoA that inhibits the cycle as well. Hormones also play a key, longer term role in the regulation of fatty acid synthesis and degradation (see below). Acetyl-CoA is also required for production of the neurotransmitter acetylcholine (see below and Chapter 19).
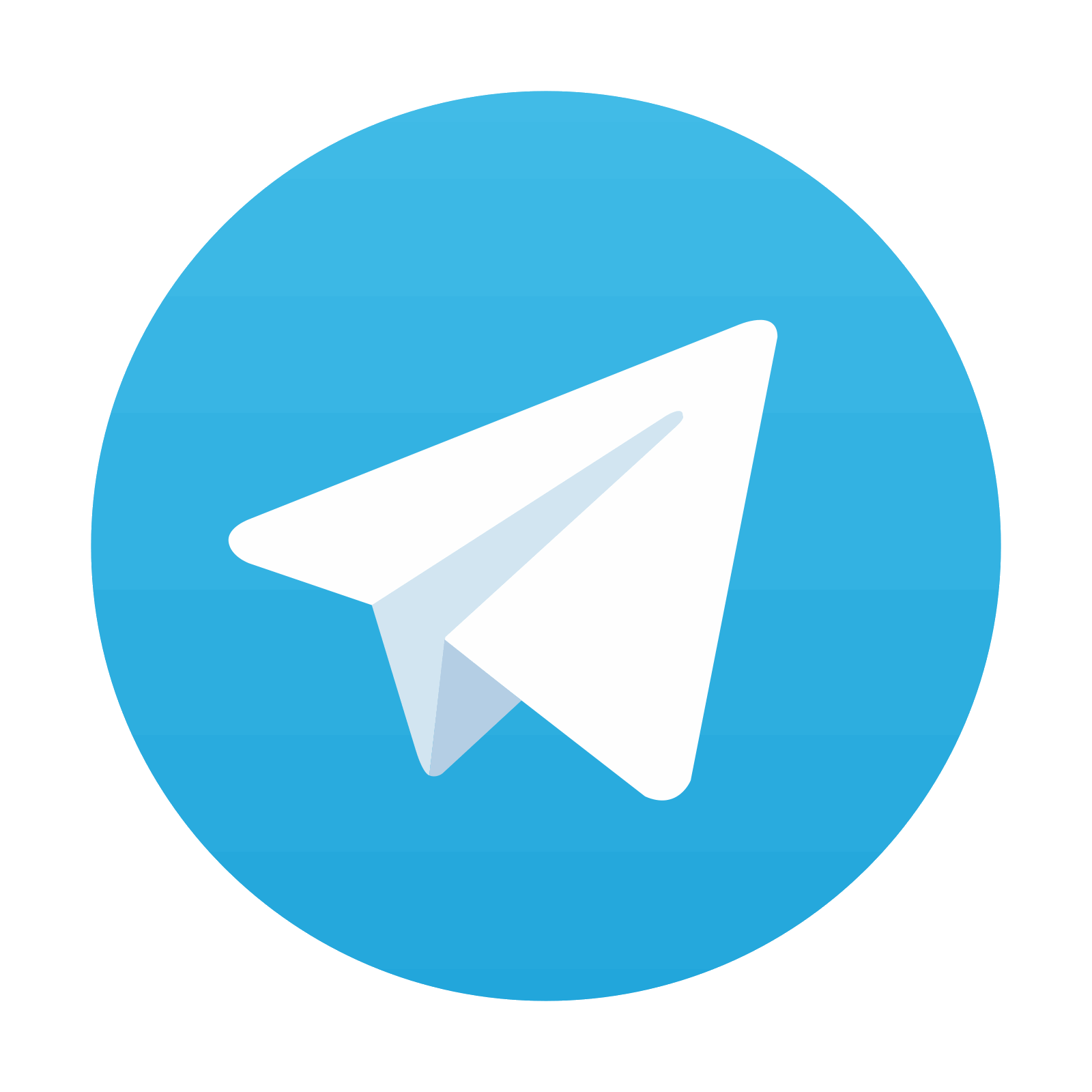
Stay updated, free articles. Join our Telegram channel
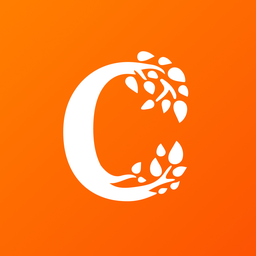
Full access? Get Clinical Tree
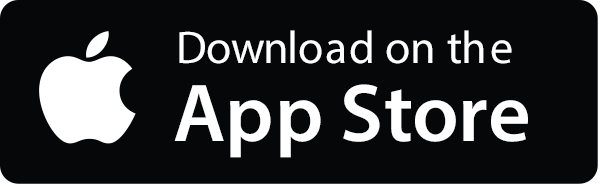
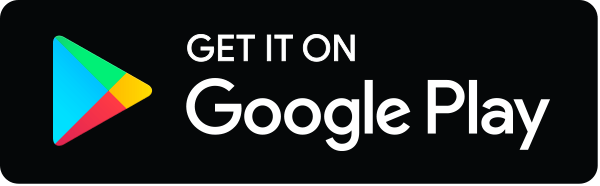