4 Young-Jae Nam1 and Nikhil Munshi2 1Department of Medicine, Division of Cardiovascular Medicine, Vanderbilt University, USA 2Internal Medicine, University of Texas Southwestern Medical Center, USA Despite remarkable advances in cardiovascular medicine, ischemic heart disease, including myocardial infarction and subsequent heart failure, is the number one cause of morbidity and mortality worldwide [1]. The fundamental yet unresolved problem is that the adult heart irreversibly loses millions to billions of cardiomyocytes during myocardial infarction due to an inability to regenerate cardiomyocytes [2]. Injured and aging cardiomyocytes are replaced by fibrotic scar. This leads to loss of pump function and provides a substrate for life-threatening arrhythmias, a common mode of death in heart failure. Thus, the ability to generate new cardiomyocytes remains an imperative scientific focus. One approach under active investigation is to transplant stem/progenitor cells, which have the potential to differentiate into cardiomyocytes, to repair the injured heart. One obvious potential source for this approach is human embryonic stem cells (ESCs), which can efficiently differentiate into cardiomyocytes and have the potential to regenerate the heart [3]. Besides ethical issues, however, two major problems limit this strategy from clinical application: immunological rejection (because ESCs are foreign to the body) and teratoma formation from undifferentiated cells. An alternative approach is to identify stem/progenitor cells that can differentiate into cardiomyocytes as the source for cell therapy. Although this approach has been extensively tested in animal models and numerous clinical trials over the past decade, clinical efficacy has been modest at best and is not supported by clear mechanistic insights [4]. Furthermore, transdifferentiation of transplanted stem/progenitor cells into cardiomyocytes has not been clearly and consistently demonstrated. Rather, it has been a general consensus that the beneficial effect of cell-based therapy results from paracrine effects of transplanted cells [5]. Induced pluripotent stem cells (iPSCs) or iPSC-derived cardiomyocytes are another attractive cell source for cell-replacement therapy and heart repair [6]. There is no doubt that iPSC reprogramming, followed by directed differentiation, offers a powerful approach to regenerating any cell type and permits in vitro production of personalized cell therapies. Before this strategy can be translated into therapeutic application, however, tumor formation from undifferentiated cells and unexpected immunogenicity must be resolved [7]. Poor survival rate of transplanted cells is another obstacle preventing clinical use of cell replacement-based strategies. As few as ∼15% of transplanted cells remain in the heart without leaking from the injection site or entering systemic circulation [8], with only 10% of the 15% surviving more than a week in the heart [9]. Overall, cell transplantation-based therapy for cardiac repair will remain inefficient unless the delivery systems and survival of transplanted cells improve. Therefore, there is a major need to develop an entirely new therapeutic strategy that will eliminate the obstacles facing current cell-based therapies for post-heart attack intervention. More than half of the cells in the heart are fibroblasts. They are activated during injury and lead to cardiac fibrosis and scar formation, thereby impeding contractility, and contribute to adverse remodeling and conduction abnormalities. Therefore, directly targeting cardiac fibroblasts in the heart so that they transform into new cardiac muscle tissue is a particularly attractive strategy for heart repair after injury (Figure 4.1). In fact, this direct phenotypic conversion from one fully differentiated cell type into another has long been described in studies, but it was previously restricted to related cell types for which a single master transcription factor controls cell fate. Examples include (i) fibroblasts to myofibers, (ii) fibroblasts to smooth muscle cells, (iii) B lymphocytes to macrophages, and (iv) inner-ear support cells to hair cells [10–13]. The discovery that a combination of transcription factors was required to reprogram iPSCs, rather than a single master transcription factor, opened up new avenues for specific lineage conversions. As a result, lineage reprogramming has been extended to other medically useful cell types that require more complex transcriptional regulation to establish their identities, including pancreatic β-cells, neurons, hepatocytes, and cardiomyocytes [14–18]. Figure 4.1 Heart repair by reprogramming of non-myocytes into cardiomyocyte-like cells in vivo. Immediately after left anterior descending (LAD) coronary artery ligation to induce myocardial infarction, a viral cocktail of reprogramming factors is directly injected into the border zone adjacent to the infarcted myocardium. The forced expression of reprogramming factors in non-myocytes in the heart after myocardial infarction induces new cardiomyocyte-like cells and leads to the improvement of contractile function and reduction of scar formation. LV, left ventricle. (Adapted by permission from Macmillan Publishers Ltd: Nature Medicine [20], copyright (2013)) A heart-repair strategy using direct reprogramming has multiple advantages over current stem cell-based approaches. First, there is no need for ex vivo manipulation of cells and reintroduction into the heart, since reprogramming occurs in vivo [19,20]. This eliminates the obstacles associated with efficient transplantation and integration of engrafted cells into the functioning myocardium. Second, this lineage reprogramming approach does not transverse a stem cell-like state, which reduces the likelihood of teratoma formation from undifferentiated cells. Third, this strategy is expected to reduce cardiac fibrosis by directly targeting cardiac fibroblasts, the principal mediators of cardiac fibrosis and scar formation after myocardial infarction (MI [21]. Fibrotic scar formation at the site of an infarct and interstitial fibrosis of adjacent myocardium act as additional barriers to cardiac repair and contribute to loss of pump function, pathological remodeling, and susceptibility to arrhythmias [22]. Fibroblasts can be induced to become skeletal and smooth muscle cells by ectopic expression of a single transcription factor, such as MyoD and Myocardin, respectively; however, a master transcription factor has not been identified for cardiac muscle despite exhaustive searches over the past 2 decades [10,11]. It is clear that cardiac cell fate is determined by a complex network of core cardiac transcription factors rather than a single transcription factor [23]. Based on this knowledge, Srivastava’s group made an important first step toward direct cardiac reprogramming. Ieda et al. first reported the ability of exogenous transcription factors to direct mouse fibroblasts toward a cardiac fate [18]. First, they identified 14 candidate transcription factors that are important in heart development. Then they created a cardiac-specific αMHC-GFP transgenic mouse to isolate neonatal cardiac fibroblasts. Using these reagents, they identified three transcription factors – Gata4, Mef2c, and Tbx5 (also known as GMT) – that were sufficient to induce cardiac phenotype in mouse fibroblasts. However, the reprogramming efficiency remains low; 5–15% of fibroblasts express cardiac markers, and only 0.5% of cardiac marker-expressing cells contract. The Olson group advanced this reprogramming protocol, showing that inclusion of another cardiac transcription factor, Hand2 (H), in the GMT cocktail significantly increases the reprogramming efficiency. Song et al. demonstrated in side-by-side comparisons that GHMT is around 4-fold more effective than GMT in cardiac reprogramming in vitro. Importantly, they focused on adult fibroblasts (cardiac and tail–tip), which are less susceptible to reprogramming than immature cell types and more likely to represent the substrate for in vivo therapeutic reprogramming [20]. The use of adult fibroblasts for cardiac reprogramming also diminishes the likelihood that residual embryonic cardiac progenitors present in neonatal preparations might contaminate fibroblast preparations. The enhanced reprogramming power of GHMT is further demonstrated by its ability to induce a contractile phenotype in adult tail–tip fibroblasts that is not achieved by GMT. A muscle-specific microRNA, miR-1, alone or in combination with other muscle-specific microRNAs (miR-133, -208, and -499), has also been reported to activate cardiac gene expression in fibroblasts with low efficiency [24]. Although a microRNA-based reprogramming approach can induce global transcriptional changes favoring a cardiac phenotype, sarcomere-like structures, calcium transients, and spontaneous contraction of reprogrammed cells have not been reported. Other groups showed that different combinations of transcription factors (GMT Myocardin and GHMT Nkx2-5) are able to induce cardiac phenotype in mouse fibroblasts [25,26]. These results indicate that multiple combinations of cardiac regulators can initiate the cardiac differentiation program because they function within complex regulatory networks that involve feed-forward and autoregulatory interactions. The most important turning point for cardiac reprogramming as a potential cardiac regenerative therapy was the unexpected success of in vivo reprogramming [19,20, 24, 27]. Srivastava and Olson’s groups independently showed that in vivo reprogramming is feasible using the same reprogramming factor combinations identified in in vitro reprogramming (GMT and GHMT, respectively) [19,20]. Both groups used retroviruses to induce reprogramming of activated cardiac fibroblasts in the infarct zone of mouse infarct models, because retroviruses infect only proliferating fibroblasts rather than quiescent cardiomyocytes. To identify the origin of newly generated cardiomyocytes, both groups performed lineage-tracing experiments using FSP1 Cre or Periostin Cre/Rosa26LacZ mice, in which they could label fibroblasts with β-galactosidase following injury. There has been concern that cardiac injury or viral transduction might unintentionally activate the promoter of these reporters. To exclude this possibility, the Olson group generated a new inducible lineage marker for noncardiomyocytes, in which MerCreMer was knocked into the Tcf21 locus by homologous recombination. Tcf21 is specific for non-myocytes within the heart, with no expression in cardiomyocytes. Using this new inducible non-myocyte lineage marker, they substantiated their conclusion that newly generated cardiomyocytes originate from non-myocytes. However, it is still unclear whether the new myocytes were derived only from cardiac fibroblasts, rather than from other non-myocyte cell types, because a true fibroblast-specific lineage marker is unavailable. Thus, these studies cannot exclude the contribution of the other reprogrammed cell types to cardiac repair by GMT or GHMT. Interestingly, the reprogramming efficiency in vivo seems to be higher than that in vitro. This result suggests that the in vivo
Challenges and New Directions for Cardiac Reprogramming
4.1 Introduction
4.2 Strategies for Heart Repair
4.3 Direct Reprogramming Approaches
Stay updated, free articles. Join our Telegram channel
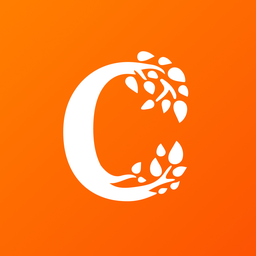
Full access? Get Clinical Tree
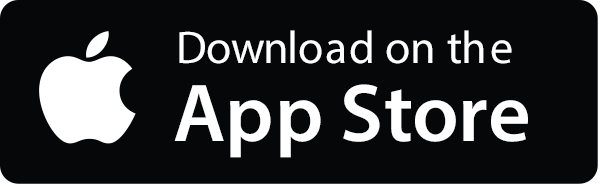
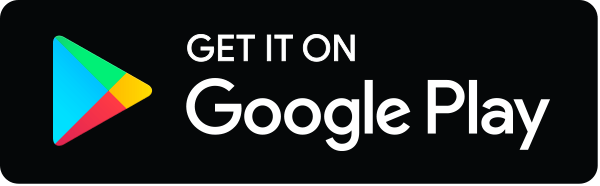