INTRODUCTION
This chapter discusses the basic structure and function of the components that make up eukaryotic and prokaryotic cells. The chapter begins with a discussion of the microscope. Historically, the microscope first revealed the presence of bacteria and later the secrets of cell structure. Today it remains a powerful tool in cell biology.
OPTICAL METHODS
The resolving power of the light microscope under ideal conditions is about half the wavelength of the light being used. (Resolving power is the distance that must separate two point sources of light if they are to be seen as two distinct images.) With yellow light of a wavelength of 0.4 μm, the smallest separable diameters are thus about 0.2 μm (ie, one-third the width of a typical prokaryotic cell). The useful magnification of a microscope is the magnification that makes visible the smallest resolvable particles. Several types of light microscopes, which are commonly used in microbiology are discussed as follows.
The bright-field microscope is most commonly used in microbiology courses and consists of two series of lenses (objective and ocular lens), which function together to resolve the image. These microscopes generally employ a 100-power objective lens with a 10-power ocular lens, thus magnifying the specimen 1000 times. Particles 0.2 μm in diameter are therefore magnified to about 0.2 mm and so become clearly visible. Further magnification would give no greater resolution of detail and would reduce the visible area (field).
With this microscope, specimens are rendered visible because of the differences in contrast between them and the surrounding medium. Many bacteria are difficult to see well because of their lack of contrast with the surrounding medium. Dyes (stains) can be used to stain cells or their organelles and increase their contrast so they can be more easily seen in the bright-field microscope.
The phase contrast microscope was developed to improve contrast differences between cells and the surrounding medium, making it possible to see living cells without staining them; with bright-field microscopes, killed and stained preparations must be used. The phase contrast microscope takes advantage of the fact that light waves passing through transparent objects, such as cells, emerge in different phases depending on the properties of the materials through which they pass. This effect is amplified by a special ring in the objective lens of a phase contrast microscope, leading to the formation of a dark image on a light background.
The dark-field microscope is a light microscope in which the lighting system has been modified to reach the specimen from the sides only. This is accomplished through the use of a special condenser that both blocks direct light rays and deflects light off a mirror on the side of the condenser at an oblique angle. This creates a “dark field” that contrasts against the highlighted edge of the specimens and results when the oblique rays are reflected from the edge of the specimen upward into the objective of the microscope. Resolution by dark-field microscopy is quite high. Thus, this technique has been particularly useful for observing organisms such as Treponema pallidum, a spirochete that is smaller than 0.2 μm in diameter and therefore cannot be observed with a bright-field or phase contrast microscope (Figure 2-1A).
FIGURE 2-1
A: Positive dark-field examination. Treponemes are recognizable by their characteristic corkscrew shape and deliberate forward and backward movement with rotation about the longitudinal axis. (Reproduced with permission. © Charles Stratton/Visuals Unlimited.) B: Fluorescence photomicrograph. A rod-shaped bacterium tagged with a fluorescent marker. (© Evans Roberts.) C: Scanning electron microscope of bacteria—Staphylococcus aureus (32,000×). (Reproduced with permission from David M. Phillips/Photo Researchers, Inc.)
The fluorescence microscope is used to visualize specimens that fluoresce, which is the ability to absorb short wavelengths of light (ultraviolet) and give off light at a longer wavelength (visible). Some organisms fluoresce naturally because of the presence within the cells of naturally fluorescent substances such as chlorophyll. Those that do not naturally fluoresce may be stained with a group of fluorescent dyes called fluorochromes. Fluorescence microscopy is widely used in clinical diagnostic microbiology. For example, the fluorochrome auramine O, which glows yellow when exposed to ultraviolet light, is strongly absorbed by Mycobacterium tuberculosis, the bacterium that causes tuberculosis. When the dye is applied to a specimen suspected of containing M tuberculosis and exposed to ultraviolet light, the bacterium can be detected by the appearance of bright yellow organisms against a dark background.
The principal use of fluorescence microscopy is a diagnostic technique called the fluorescent-antibody (FA) technique or immunofluorescence. In this technique, specific antibodies (eg, antibodies to Legionella pneumophila) are chemically labeled with a fluorochrome such as fluorescein isothiocyanate (FITC). These fluorescent antibodies are then added to a microscope slide containing a clinical specimen. If the specimen contains L pneumophila, the fluorescent antibodies will bind to antigens on the surface of the bacterium, causing it to fluoresce when exposed to ultraviolet light (Figure 2-1B).
Differential interference contrast (DIC) microscopes employ a polarizer to produce polarized light. The polarized light beam passes through a prism that generates two distinct beams; these beams pass through the specimen and enter the objective lens, where they are recombined into a single beam. Because of slight differences in refractive index of the substances each beam passed through, the combined beams are not totally in phase but instead create an interference effect, which intensifies subtle differences in cell structure. Structures such as spores, vacuoles, and granules appear three-dimensional. DIC microscopy is particularly useful for observing unstained cells because of its ability to generate images that reveal internal cell structures that are less apparent by bright-field techniques.
The high resolving power of electron microscopes has enabled scientists to observe the detailed structures of prokaryotic and eukaryotic cells. The superior resolution of the electron microscope is due to the fact that electrons have a much shorter wavelength than the photons of white light.
There are two types of electron microscopes in general use: The transmission electron microscope (TEM), which has many features in common with the light microscope, and the scanning electron microscope (SEM). The TEM was the first to be developed and uses a beam of electrons projected from an electron gun and directed or focused by an electromagnetic condenser lens onto a thin specimen. As the electrons strike the specimen, they are differentially scattered by the number and mass of atoms in the specimen; some electrons pass through the specimen and are gathered and focused by an electromagnetic objective lens, which presents an image of the specimen to the projector lens system for further enlargement. The image is visualized by allowing it to impinge on a screen that fluoresces when struck with the electrons. The image can be recorded on photographic film. TEM can resolve particles 0.001 μm apart. Viruses with diameters of 0.01–0.2 μm can be easily resolved.
The SEM generally has a lower resolving power than the TEM; however, it is particularly useful for providing three-dimensional images of the surface of microscopic objects. Electrons are focused by means of lenses into a very fine point. The interaction of electrons with the specimen results in the release of different forms of radiation (eg, secondary electrons) from the surface of the material, which can be captured by an appropriate detector, amplified, and then imaged on a television screen (Figure 2-1C).
An important technique in electron microscopy is the use of “shadowing.” This involves depositing a thin layer of heavy metal (eg, platinum) on the specimen by placing it in the path of a beam of metal ions in a vacuum. The beam is directed at a low angle to the specimen so that it acquires a “shadow” in the form of an uncoated area on the other side. When an electron beam is then passed through the coated preparation in the electron microscope and a positive print is made from the “negative” image, a three-dimensional effect is achieved (eg, see Figure 2-21).
Other important techniques in electron microscopy include the use of ultrathin sections of embedded material, a method of freeze-drying specimens that prevents the distortion caused by conventional drying procedures, and the use of negative staining with an electron-dense material such as phosphotungstic acid or uranyl salts (eg, see Figure 42-1). Without these heavy metal salts, there would not be enough contrast to detect the details of the specimen.
The confocal scanning laser microscope (CSLM) couples a laser light source to a light microscope. In confocal scanning laser microscopy, a laser beam is bounced off a mirror that directs the beam through a scanning device. Then the laser beam is directed through a pinhole that precisely adjusts the plane of focus of the beam to a given vertical layer within the specimen. By precisely illuminating only a single plane of the specimen, illumination intensity drops off rapidly above and below the plane of focus, and stray light from other planes of focus are minimized. Thus, in a relatively thick specimen, various layers can be observed by adjusting the plane of focus of the laser beam.
Cells are often stained with fluorescent dyes to make them more visible. Alternatively, false color images can be generated by adjusting the microscope in such a way as to make different layers take on different colors. The CSLM is equipped with computer software to assemble digital images for subsequent image processing. Thus, images obtained from different layers can be stored and then digitally overlaid to reconstruct a three-dimensional image of the entire specimen.
A new class of microscopes, called scanning probe microscopes, measures surface features by moving a sharp probe over the object’s surface. The scanning tunneling microscope and the atomic force microscope are examples of this new class of microscopes, which enable scientists to view atoms or molecules on the surface of a specimen. For example, interactions between proteins of the bacterium Escherichia coli can be studied with the atomic force microscope (Figure 2-2).
EUKARYOTIC CELL STRUCTURE
The nucleus contains the cell’s genome. It is bounded by a membrane that consists of a pair of unit membranes separated by a space of variable thickness. The inner membrane is usually a simple sac, but the outermost membrane is, in many places, continuous with the endoplasmic reticulum (ER). The nuclear membrane exhibits selective permeability because of pores, which consist of a complex of several proteins whose function is to import substances into and export substances out of the nucleus. The chromosomes of eukaryotic cells contain linear DNA macromolecules arranged as a double helix. They are only visible with a light microscope when the cell is undergoing division and the DNA is in a highly condensed form; at other times, the chromosomes are not condensed and appear as in Figure 2-3. Eukaryotic DNA macromolecules are associated with basic proteins called histones that bind to the DNA by ionic interactions.
FIGURE 2-3
Eukaryotic cells. A: Diagrammatic representation of an animal cell. B: Diagrammatic representation of a plant cell. C: Micrograph of an animal cell shows several membrane-bound structures, including mitochondria and a nucleus. (Fig. 2-3(A) and (B) Reproduced with permission from Nester EW, Anderson DG, Roberts CE, Nester MT: Microbiology: A Human Perspective, 6th ed. McGraw-Hill, 2009. Fig. 2-3(C) Reproduced with permission from Thomas Fritsche, MD, PhD.)
A structure often visible within the nucleus is the nucleolus, an area rich in RNA that is the site of ribosomal RNA synthesis (see Figure 2-3). Ribosomal proteins synthesized in the cytoplasm are transported into the nucleolus and combine with ribosomal RNA to form the small and large subunits of the eukaryotic ribosome. These are then exported to the cytoplasm, where they associate to form an intact ribosome that can function in protein synthesis.
The cytoplasm of eukaryotic cells is characterized by the presence of an ER, vacuoles, self-reproducing plastids, and an elaborate cytoskeleton composed of microtubules, microfilaments, and intermediate filaments.
The endoplasmic reticulum (ER) is a network of membrane-bound channels continuous with the nuclear membrane. Two types of ER are recognized: rough, which contains attached 80S ribosomes, and smooth, which does not (see Figure 2-3). Rough ER is a major producer of glycoproteins and produces new membrane material that is transported throughout the cell; smooth ER participates in the synthesis of lipids and in some aspects of carbohydrate metabolism. The Golgi complex consists of a stack of membranes that function in concert with the ER to chemically modify and sort products of the ER into those destined to be secreted and those that function in other membranous structures of the cell.
The plastids include mitochondria and chloroplasts. Several lines of evidence suggest that mitochondria and chloroplasts were descendents of ancient prokaryotic organisms and arose from the engulfment of a prokaryotic cell by a larger cell (endosymbiosis). Mitochondria are of prokaryotic size, and its membrane, which lacks sterols, is much less rigid than the eukaryotic cell’s cytoplasmic membrane, which does contain sterols. Mitochondria contain two sets of membranes. The outermost membrane is rather permeable, having numerous minute channels that allow passage of ions and small molecules (eg, adenosine triphosphate [ATP]). Invagination of the outer membrane forms a system of inner folded membranes called cristae. The cristae are the sites of enzymes involved in respiration and ATP production. Cristae also contain specific transport proteins that regulate passage of metabolites into and out of the mitochondrial matrix. The matrix contains a number of enzymes, particularly those of the citric acid cycle. Chloroplasts are photosynthetic cell organelles that are capable of converting the energy of sunlight into chemical energy through photosynthesis. Chlorophyll and all other components needed for photosynthesis are located in a series of flattened membrane discs called thylakoids. The size, shape, and number of chloroplasts per cell vary markedly; in contrast to mitochondria, chloroplasts are generally much larger than prokaryotes. Mitochondria and chloroplasts contain their own DNA, which exists in a covalently closed circular form and codes for some (not all) of their constituent proteins and transfer RNAs. Mitochondria and chloroplasts also contain 70S ribosomes, the same as those of prokaryotes.
Some eukaryotic microorganisms (eg, Trichomonas vaginalis) lack mitochondria and contain instead a membrane-enclosed respiratory organelle called the hydrogenosome. Hydrogenosomes may have arisen by endosymbiosis, and some have been identified that contain DNA and ribosomes. The hydrogenosome, although similar in size to mitochondria, lacks cristae and the enzymes of the tricarboxylic acid cycle. Pyruvate is taken up by the hydrogenosome, and H2, CO2, acetate, and ATP are produced.
Lysosomes are membrane-enclosed sacs that contain various digestive enzymes that the cell uses to digest macromolecules such as proteins, fats, and polysaccharides. The lysosome allows these enzymes to be partitioned away from the cytoplasm proper, where they could destroy key cellular macromolecules if not contained. After the hydrolysis of macromolecules in the lysosome, the resulting monomers pass from the lysosome into the cytoplasm, where they serve as nutrients.
The peroxisome is a membrane-enclosed structure whose function is to produce H2O2 from the reduction of O2 by various hydrogen donors. The H2O2 produced in the peroxisome is subsequently degraded to H2O and O2 by the enzyme catalase.
The cytoskeleton is a three-dimensional structure that fills the cytoplasm. The primary types of fibers comprising the cytoskeleton are microfilaments, intermediate filaments, and microtubules. Microfilaments are about 3–6 nm in diameter and are polymers composed of subunits of the protein actin. These fibers form scaffolds throughout the cell, defining and maintaining the shape of the cell. Microfilaments can also carry out cellular movements, including gliding, contraction, and cytokinesis.
Microtubules are cylindrical tubes 20–25 nm in diameter and are composed of subunits of the protein tubulin. Microtubules assist microfilaments in maintaining cell structure, form the spindle fibers for separating chromosomes during mitosis, and play an important role in cell motility. Intermediate filaments are about 10 nm in diameter and provide tensile strength for the cell.
The cytoplasm is enclosed within a plasma membrane composed of protein and phospholipid similar to the prokaryotic cell membrane illustrated later (see Figure 2-11). Most animal cells have no other surface layers; however, plant cells have an outer cell wall composed of cellulose (Figure 2-3b). Many eukaryotic microorganisms also have an outer cell wall, which may be composed of a polysaccharide such as cellulose or chitin or may be inorganic (eg, the silica wall of diatoms).
Many eukaryotic microorganisms have organelles called flagella (eg, T vaginalis) or cilia (eg, Paramecium) that move with a wavelike motion to propel the cell through water. Eukaryotic flagella emanate from the polar region of the cell, and cilia, which are shorter than flagella, surround the cell (Figure 2-4). Both the flagella and the cilia of eukaryotic cells have the same basic structure and biochemical composition. Both consist of a series of microtubules, hollow protein cylinders composed of a protein called tubulin surrounded by a membrane. The arrangement of the microtubules is called the “9 + 2 system” because it consists of nine peripheral pairs of microtubules surrounding two single central microtubules (Figure 2-5).
FIGURE 2-5
Cilia and flagella structure. A: An electron micrograph of a cilium cross section. Note the two central microtubles surrounded by nine microtubule doublets (160,000×). (Reproduced with permission. © KG Murti/Visuals Unlimited.) B: A diagram of cilia and flagella structure. (Reproduced with permission from Willey JM, Sherwood LM, Woolverton CJ [editors]: Prescott, Harley, and Klein’s Microbiology, 7th ed. McGraw-Hill; 2008. © The McGraw-Hill Companies, Inc.)
PROKARYOTIC CELL STRUCTURE
The prokaryotic cell is simpler than the eukaryotic cell at every level, with one exception: The cell envelope is more complex.
Prokaryotes have no true nuclei; instead they package their DNA in a structure known as the nucleoid. The negatively charged DNA is at least partially neutralized by small polyamines and magnesium ions, but histone-like proteins exist in bacteria and presumably play a role similar to that of histones in eukaryotic chromatin.
Electron micrographs of a typical prokaryotic cell reveal the absence of a nuclear membrane and a mitotic apparatus. The exception to this rule is the planctomycetes, a divergent group of aquatic bacteria, which have a nucleoid surrounded by a nuclear envelope consisting of two membranes. The distinction between prokaryotes and eukaryotes that still holds is that prokaryotes have no eukaryotic-type mitotic apparatus. The nuclear region (Figure 2-6) is filled with DNA fibrils. The nucleoid of most bacterial cells consists of a single continuous circular molecule ranging in size from 0.58 to almost 10 million base pairs. However, a few bacteria have been shown to have two, three, or even four dissimilar chromosomes. For example, Vibrio cholerae and Brucella melitensis have two dissimilar chromosomes. There are exceptions to this rule of circularity because some prokaryotes (eg, Borrelia burgdorferi and Streptomyces coelicolor) have been shown to have a linear chromosome.
FIGURE 2-6
The nucleoid. A: Color-enhanced transmission electron micrograph of Escherichia coli with the DNA shown in red. (© CNRI/SPL/Photo Researchers, Inc.) B: Chromosome released from a gently lysed cell of E coli. Note how tightly packaged the DNA must be inside the bacterium. (© Dr. Gopal Murti/SPL/Photo Researchers.)
In bacteria, the number of nucleoids, and therefore the number of chromosomes, depend on the growth conditions. Rapidly growing bacteria have more nucleoids per cell than slowly growing ones; however, when multiple copies are present, they are all the same (ie, prokaryotic cells are haploid).
Prokaryotic cells lack autonomous plastids, such as mitochondria and chloroplasts; the electron transport enzymes are localized instead in the cytoplasmic membrane. The photosynthetic pigments (carotenoids, bacteriochlorophyll) of photosynthetic bacteria are contained in intracytoplasmic membrane systems of various morphologies. Membrane vesicles (chromatophores) or lamellae are commonly observed membrane types. Some photosynthetic bacteria have specialized nonunit membrane-enclosed structures called chlorosomes. In some Cyanobacteria (formerly known as blue-green algae), the photosynthetic membranes often form multilayered structures known as thylakoids (Figure 2-7). The major accessory pigments used for light harvesting are the phycobilins found on the outer surface of the thylakoid membranes.
Bacteria often store reserve materials in the form of insoluble granules, which appear as refractile bodies in the cytoplasm when viewed by phase contrast microscopy. These so-called inclusion bodies almost always function in the storage of energy or as a reservoir of structural building blocks. Most cellular inclusions are bounded by a thin nonunit membrane consisting of lipid, which serves to separate the inclusion from the cytoplasm proper. One of the most common inclusion bodies consists of poly-β-hydroxybutyric acid (PHB), a lipid-like compound consisting of chains of β-hydroxybutyric acid units connected through ester linkages. PHB is produced when the source of nitrogen, sulfur, or phosphorous is limited and there is excess carbon in the medium (Figure 2-8A). Another storage product formed by prokaryotes when carbon is in excess is glycogen, which is a polymer of glucose. PHB and glycogen are used as carbon sources when protein and nucleic acid synthesis are resumed. A variety of prokaryotes are capable of oxidizing reduced sulfur compounds such as hydrogen sulfide and thiosulfate, producing intracellular granules of elemental sulfur (Figure 2-8B). As the reduced sulfur source becomes limiting, the sulfur in the granules is oxidized, usually to sulfate, and the granules slowly disappear. Many bacteria accumulate large reserves of inorganic phosphate in the form of granules of polyphosphate. These granules can be degraded and used as sources of phosphate for nucleic acid and phospholipid synthesis to support growth. These granules are sometimes termed volutin granules or metachromatic granules because they stain red with a blue dye. They are characteristic features of the corynebacteria (see Chapter 13).
FIGURE 2-8
Inclusion bodies in bacteria. A: Electron micrograph of Bacillus megaterium (30,500×) showing poly-β-hydroxybutyric acid inclusion body, PHB; cell wall, CW; nucleoid, N; plasma membrane, PM; “mesosome,” M; and ribosomes, R. (Reproduced with permission. © Ralph A. Slepecky/Visuals Unlimited.) B: Cromatium vinosum, a purple sulfur bacterium, with intracellular sulfur granules, bright field microscopy (2000×). (Reproduced with permission from Holt J (editor): The Shorter Bergey’s Manual of Determinative Bacteriology, 8th ed. Williams & Wilkins, 1977. Copyright Bergey’s Manual Trust.)
Certain groups of autotrophic bacteria that fix carbon dioxide to make their biochemical building blocks contain polyhedral bodies surrounded by a protein shell (carboxysomes) containing the key enzyme of CO2 fixation, ribulosebisphosphate carboxylase (see Figure 2-7). Magnetosomes are intracellular crystal particles of the iron mineral magnetite (Fe3O4) that allow certain aquatic bacteria to exhibit magnetotaxis (ie, migration or orientation of the cell with respect to the earth’s magnetic field). Magnetosomes are surrounded by a nonunit membrane containing phospholipids, proteins, and glycoproteins. Gas vesicles are found almost exclusively in microorganisms from aquatic habitats, where they provide buoyancy. The gas vesicle membrane is a 2-nm-thick layer of protein, impermeable to water and solutes but permeable to gases; thus, gas vesicles exist as gas-filled structures surrounded by the constituents of the cytoplasm (Figure 2-9).
Bacteria contain proteins resembling both the actin and nonactin cytoskeletal proteins of eukaryotic cells as additional proteins that play cytoskeletal roles (Figure 2-10). Actin homologs (eg, MreB, Mbl) perform a variety of functions, helping to determine cell shape, segregate chromosomes, and localize proteins with the cell. Nonactin homologs (eg, FtsZ) and unique bacterial cytoskeletal proteins (eg, SecY, MinD) are involved in determining cell shape and in regulation of cell division and chromosome segregation.
FIGURE 2-10
The prokaryotic cytoskeleton. Visualization of the MreB-like cytoskeletal protein (Mbl) of Bacillus subtilis. The Mbl protein has been fused with green fluorescent protein, and live cells have been examined by fluorescence microscopy. A: Arrows point to the helical cytoskeleton cables that extend the length of the cells. B: Three of the cells from A are shown at a higher magnification. (Courtesy of Rut Carballido-Lopez and Jeff Errington.)
Prokaryotic cells are surrounded by complex envelope layers that differ in composition among the major groups. These structures protect the organisms from hostile environments, such as extreme osmolarity, harsh chemicals, and even antibiotics.
The bacterial cell membrane, also called the cytoplasmic membrane, is visible in electron micrographs of thin sections (see Figure 2-15). It is a typical “unit membrane” composed of phospholipids and upward of 200 different kinds of proteins. Proteins account for approximately 70% of the mass of the membrane, which is a considerably higher proportion than that of mammalian cell membranes. Figure 2-11 illustrates a model of membrane organization. The membranes of prokaryotes are distinguished from those of eukaryotic cells by the absence of sterols, the only exception being mycoplasmas that incorporate sterols, such as cholesterol, into their membranes when growing in sterol-containing media.
FIGURE 2-11
Bacterial plasma membrane structure. This diagram of the fluid mosaic model of bacterial membrane structure shown the integral proteins (green and red) floating in a lipid bilayer. Peripheral proteins (yellow) are associated loosely with the inner membrane surface. Small spheres represent the hydrophilic ends of membrane phospholipids and wiggly tails, the hydrophobic fatty acid chains. Other membrane lipids such as hopanoids (purple) may be present. For the sake of clarity, phospholipids are shown proportionately much larger size than in real membranes. (Reproduced with permission from Willey JM, Sherwood LM, Woolverton CJ [editors]: Prescott, Harley, and Klein’s Microbiology, 7th ed. McGraw-Hill; 2008. © The McGraw-Hill Companies, Inc.)
The cell membranes of the Archaea (see Chapter 1) differ from those of the Bacteria. Some Archaeal cell membranes contain unique lipids, isoprenoids, rather than fatty acids, linked to glycerol by ether rather than an ester linkage. Some of these lipids have no phosphate groups, and therefore, they are not phospholipids. In other species, the cell membrane is made up of a lipid monolayer consisting of long lipids (about twice as long as a phospholipid) with glycerol ethers at both ends (diglycerol tetraethers). The molecules orient themselves with the polar glycerol groups on the surfaces and the nonpolar hydrocarbon chain in the interior. These unusual lipids contribute to the ability of many Archaea to grow under environmental conditions such as high salt, low pH, or very high temperature.
The major functions of the cytoplasmic membrane are (1) selective permeability and transport of solutes; (2) electron transport and oxidative phosphorylation in aerobic species; (3) excretion of hydrolytic exoenzymes; (4) bearing the enzymes and carrier molecules that function in the biosynthesis of DNA, cell wall polymers, and membrane lipids; and (5) bearing the receptors and other proteins of the chemotactic and other sensory transduction systems.
At least 50% of the cytoplasmic membrane must be in the semifluid state for cell growth to occur. At low temperatures, this is achieved by greatly increased synthesis and incorporation of unsaturated fatty acids into the phospholipids of the cell membrane.
1. Permeability and transport—The cytoplasmic membrane forms a hydrophobic barrier impermeable to most hydrophilic molecules. However, several mechanisms (transport systems) exist that enable the cell to transport nutrients into and waste products out of the cell. These transport systems work against a concentration gradient to increase the concentration of nutrients inside the cell, a function that requires energy in some form. There are three general transport mechanisms involved in membrane transport: passive transport, active transport, and group translocation.
a. Passive transport—This mechanism relies on diffusion, uses no energy, and operates only when the solute is at higher concentration outside than inside the cell. Simple diffusion accounts for the entry of very few nutrients, including dissolved oxygen, carbon dioxide, and water itself. Simple diffusion provides neither speed nor selectivity. Facilitated diffusion also uses no energy so the solute never achieves an internal concentration greater than what exists outside the cell. However, facilitated diffusion is selective. Channel proteins form selective channels that facilitate the passage of specific molecules. Facilitated diffusion is common in eukaryotic microorganisms (eg, yeast) but is rare in prokaryotes. Glycerol is one of the few compounds that enters prokaryotic cells by facilitated diffusion.
b. Active transport—Many nutrients are concentrated more than a thousand-fold as a result of active transport. There are two types of active transport mechanisms depending on the source of energy used: ion-coupled transport and ATP-binding cassette (ABC) transport.
1) Ion-coupled transport—These systems move a molecule across the cell membrane at the expense of a previously established ion gradient such as protonmotive or sodium-motive force. There are three basic types: uniport, symport, and antiport (Figure 2-12). Ion-coupled transport is particularly common in aerobic organisms, which have an easier time generating an ion-motive force than do anaerobes. Uniporters catalyze the transport of a substrate independent of any coupled ion. Symporters catalyze the simultaneous transport of two substrates in the same direction by a single carrier; for example, an H+ gradient can permit symport of an oppositely charged ion (eg, glycine) or a neutral molecule (eg, galactose). Antiporters catalyze the simultaneous transport of two like-charged compounds in opposite directions by a common carrier (eg, H+:Na+). Approximately 40% of the substrates transported by E coli use this mechanism.
2) ABC transport—This mechanism uses ATP directly to transport solutes into the cell. In gram-negative bacteria, the transport of many nutrients is facilitated by specific binding proteins located in the periplasmic space; in gram-positive cells, the binding proteins are attached to the outer surface of the cell membrane. These proteins function by transferring the bound substrate to a membrane-bound protein complex. Hydrolysis of ATP is then triggered, and the energy is used to open the membrane pore and allow the unidirectional movement of the substrate into the cell. Approximately 40% of the substrates transported by E coli use this mechanism.
c. Group translocation—In addition to true transport, in which a solute is moved across the membrane without change in structure, bacteria use a process called group translocation (vectorial metabolism) to effect the net uptake of certain sugars (eg, glucose and mannose), the substrate becoming phosphorylated during the transport process. In a strict sense, group translocation is not active transport because no concentration gradient is involved. This process allows bacteria to use their energy resources efficiently by coupling transport with metabolism. In this process, a membrane carrier protein is first phosphorylated in the cytoplasm at the expense of phosphoenolpyruvate; the phosphorylated carrier protein then binds the free sugar at the exterior membrane face and transports it into the cytoplasm, releasing it as sugar phosphate. Such systems of sugar transport are called phosphotransferase systems. Phosphotransferase systems are also involved in movement toward these carbon sources (chemotaxis) and in the regulation of several other metabolic pathways (catabolite repression).
d. Special transport processes—Iron (Fe) is an essential nutrient for the growth of almost all bacteria. Under anaerobic conditions, Fe is generally in the +2 oxidation state and soluble. However, under aerobic conditions, Fe is generally in the +3 oxidation state and insoluble. The internal compartments of animals contain virtually no free Fe; it is sequestered in complexes with such proteins as transferrin and lactoferrin. Some bacteria solve this problem by secreting siderophores—compounds that chelate Fe and promote its transport as a soluble complex. One major group of siderophores consists of derivatives of hydroxamic acid (−CONH2OH), which chelate Fe3+ very strongly. The iron–hydroxamate complex is actively transported into the cell by the cooperative action of a group of proteins that span the outer membrane, periplasm, and inner membrane. The iron is released, and the hydroxamate can exit the cell and be used again for iron transport.
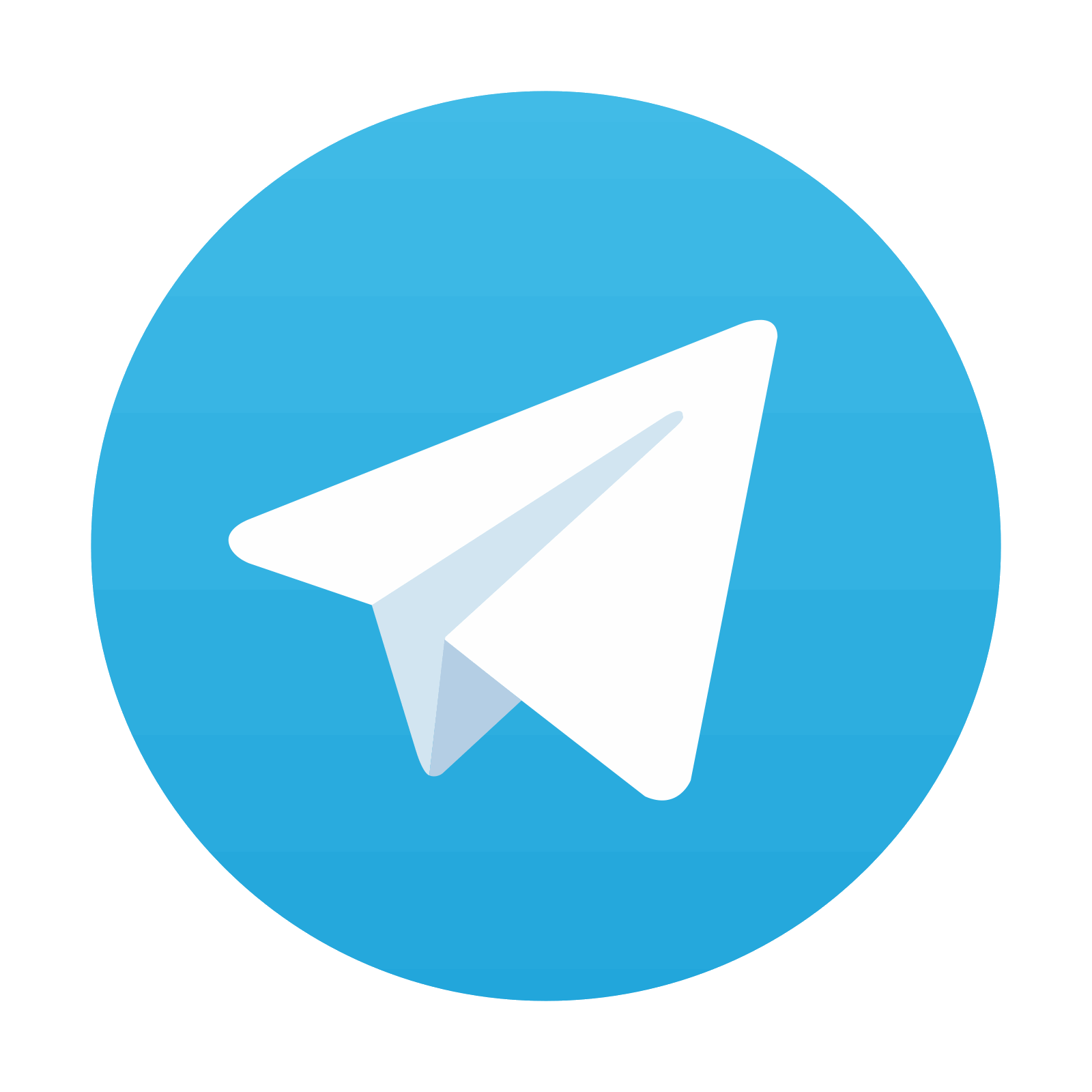
Stay updated, free articles. Join our Telegram channel
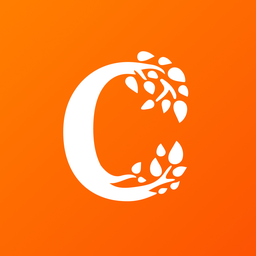
Full access? Get Clinical Tree
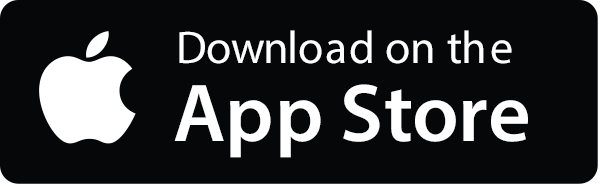
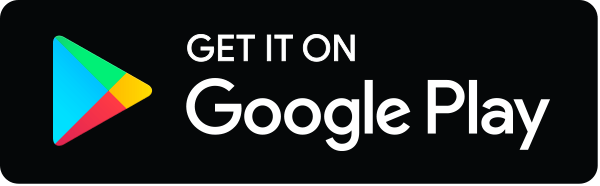