Chapter 22 Carbohydrate Metabolism
In addition to the catabolism of glucose (see Chapter 21), carbohydrate metabolism has several other functions:
An adequate blood glucose level must be maintained at all times
Gluconeogenesis bypasses the three irreversible reactions of glycolysis
The easiest strategy for glucose synthesis would be to reverse glycolysis by making glucose from pyruvate and lactate. To do so, however, the gluconeogenic pathway has to bypass the three irreversible reactions of glycolysis: those catalyzed by hexokinase, phosphofructokinase (PFK), and pyruvate kinase (Fig. 22.1).
This feat is accomplished in a sequence of two reactions (Fig. 22.2). First, pyruvate is carboxylated to oxaloacetate by pyruvate carboxylase. This ATP-dependent carboxylation reaction was described as an anaplerotic reaction of the tricarboxylic acid (TCA) cycle in Chapter 21. The second reaction, catalyzed by PEP-carboxykinase, converts oxaloacetate into PEP. It requires GTP, which supplies the phosphate group in PEP. The two reactions combined have a standard free energy change (ΔG0′) of +0.2 kcal/mol, but in the cell they proceed only from pyruvate to PEP because of the high cellular concentration ratios of [ATP]/[ADP], [GTP]/[GDP], and [pyruvate]/[PEP].
Whereas pyruvate carboxylase is strictly mitochondrial, PEP-carboxykinase is both mitochondrial and cytoplasmic. PEP is transported across the inner mitochondrial membrane, whereas oxaloacetate is shuttled into the cytoplasm after being reduced to malate or transaminated to aspartate (see Fig. 21.21).
The remaining irreversible reactions of glycolysis, catalyzed by PFK and hexokinase, are bypassed by the hydrolytic removal of phosphate. Fructose-1,6-bisphosphatase hydrolyzes the phosphate from carbon 1 of fructose-1,6-bisphosphate (Fig. 22.3), and glucose-6-phosphatase removes the phosphate from glucose-6-phosphate. Both reactions are irreversible. Unlike the other gluconeogenic enzymes, which are cytoplasmic (except pyruvate carboxylase), glucose-6-phosphatase resides on the inner surface of the endoplasmic reticulum membrane.
Fatty acids cannot be converted into glucose
Lactate and alanine are convenient substrates of gluconeogenesis because they are readily converted to pyruvate by lactate dehydrogenase and by transamination, respectively (Fig. 22.4). Oxaloacetate is not only a gluconeogenic intermediate but also a member of the TCA cycle. This is important because most amino acids are degraded to TCA cycle intermediates. Through the TCA cycle, these “glucogenic” amino acids feed into gluconeogenesis.
Glycerol is another substrate of gluconeogenesis. It enters the pathway at the level of the triose phosphates (Fig. 22.5).
Glycolysis and gluconeogenesis are regulated by hormones
The following hormones participate in the control of hepatic glycolysis and gluconeogenesis:
The hormones regulate the synthesis of the distinctive glycolytic and gluconeogenic enzymes at the level of transcription (Fig. 22.6, A). Because this involves the synthesis of new enzyme protein and most of the enzymes have lifespans of a few days in the cell, regulation of enzyme synthesis works on a time scale of days rather than minutes.
Glycolysis and gluconeogenesis are fine tuned by allosteric effectors and hormone-induced enzyme phosphorylations
The short-term control of glycolysis and gluconeogenesis is shown in Figure 22.6, B.
This bifunctional enzyme is phosphorylated by the cAMP-activated protein kinase A in response to glucagon and is dephosphorylated in the presence of insulin. The dephosphorylated enzyme acts as a kinase that makes fructose-2,6-bisphosphate, whereas the phosphorylated form acts as a phosphatase that breaks it down (Fig. 22.7). Therefore the level of fructose-2,6-bisphosphate in the liver is high when insulin is high and glucagon is low, and the level of fructose-2,6-bisphosphate is low when insulin is low and glucagon is high. As a consequence, glycolysis is turned on when insulin is high, and gluconeogenesis is turned on when glucagon is high.
The glucose-phosphorylating enzyme in the liver is isoenzyme 4 of hexokinase, better known as glucokinase. The most important kinetic difference between glucokinase and the other isoenzymes of hexokinase is the Michaelis constant (Km) for glucose. Whereas the other forms of hexokinase have Km values near 0.1 mmol/L (2 mg/dl), glucokinase has a Km near 10 mmol/L (200 mg/dl). Glucokinase also shows a sigmoidal rather than hyperbolic relationship between glucose concentration and reaction rate (Fig. 22.8).
Carbohydrate is stored as glycogen
Glycogen is a branched polymer of between 10,000 and 40,000 glucose residues held together by α-1,4 glycosidic bonds. Approximately one in 12 glucose residues serves as a branch point by forming an α-1,6 glycosidic bond with another glucose residue (Fig. 22.9). With a molecular weight between 106 and 107 D, it is as big as a complete human ribosome (4.2 × 106 D).
Glycogen is readily synthesized from glucose
The steps in the synthesis of glycogen from glucose are outlined in Figures 22.10 and 22.11. Glucose-6-phosphate is isomerized to glucose-1-phosphate by phosphoglucomutase. There are about 20 molecules of glucose-6-phosphate for every molecule of glucose-1-phosphate at equilibrium. Glucose-1-phosphate then reacts with uridine triphosphate (UTP) to form UDP–glucose. This otherwise reversible reaction is driven to completion by the subsequent hydrolysis of pyrophosphate. UDP-glucose is the activated form of glucose for biosynthetic reactions.

Figure 22.10 Synthesis of uridine diphosphate (UDP)-glucose. UDP-glucose is the activated form of glucose for glycogen synthesis but also for the synthesis of other complex carbohydrates (see Table 8.3).
Glycogen synthase forms the α-1,4 glycosidic bonds in glycogen by transferring the glucose residue from UDP-glucose to the 4-hydroxyl group at the nonreducing end of the glycogen molecule, elongating the outer branches of glycogen by one glucose residue at a time (see Fig. 22.11).
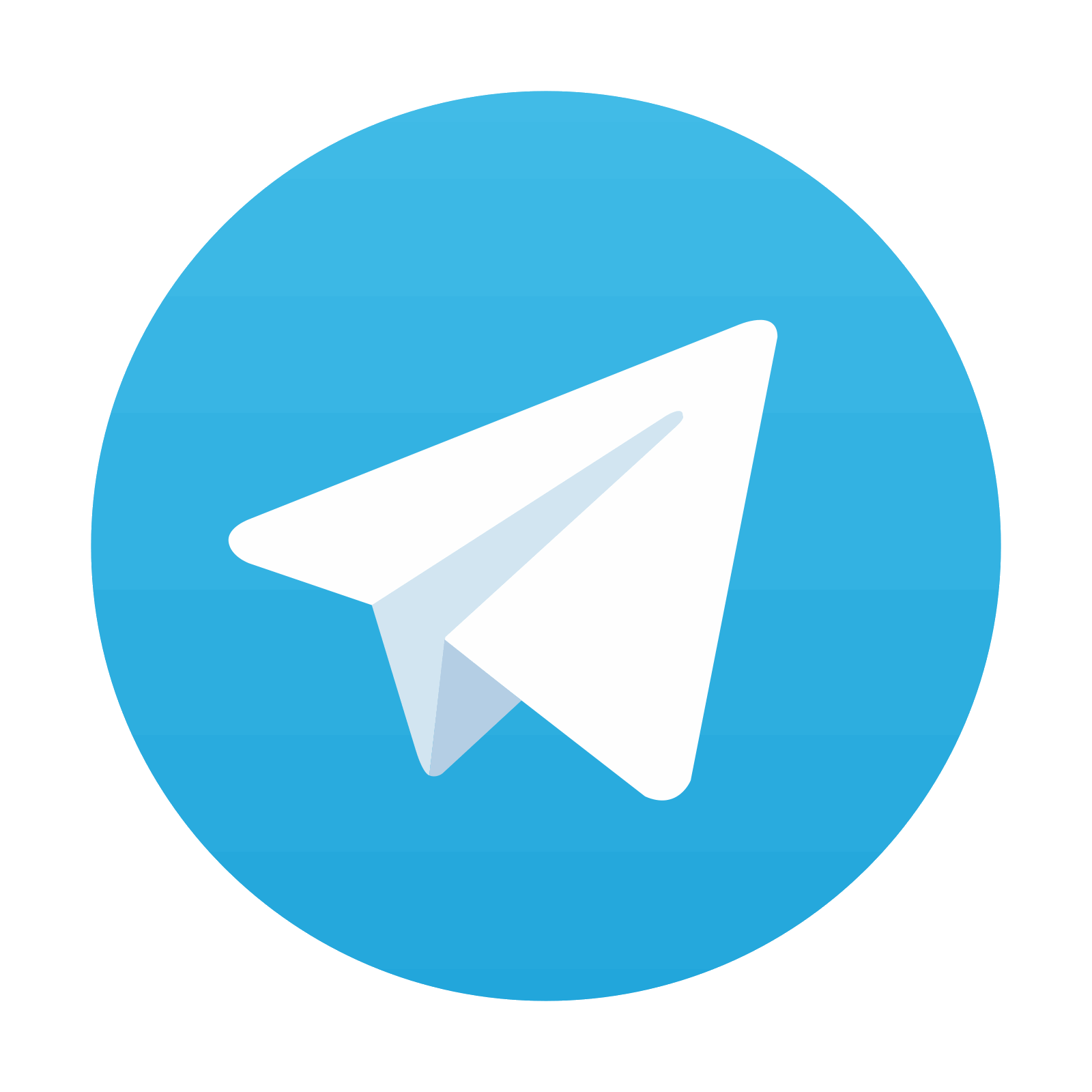
Stay updated, free articles. Join our Telegram channel
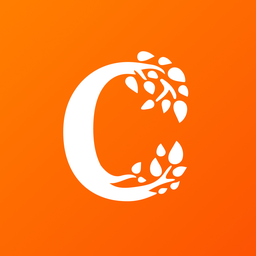
Full access? Get Clinical Tree
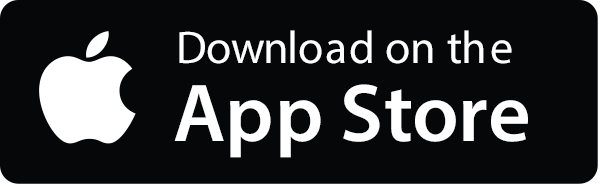
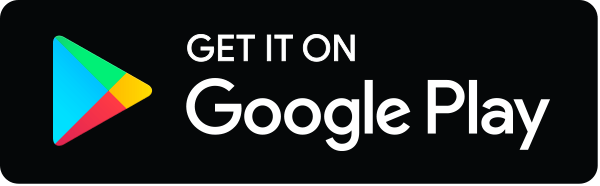