12 Causes of cancer include chemical carcinogens, radiant energy, and viruses such as the human papilloma and Epstein-Barr viruses. Factors that contribute to cancer risk include inherited genetic abnormalities, hormones, increasing age, chronic irritation, trauma and local environmental factors such as pollution and parasites. These operate in a secondary way to predispose an individual to cancer, they are not carcinogenic as such.1 It has been estimated that 70–90% of all human cancers can be attributed to environmental chemical causes (Tomatis 1979). Many of the known human carcinogens are products of the industrial revolution, such as diethylstilbestrol, benzene and asbestos. Today we are exposed to a greater number of industrial chemicals, often in complex combinations, than at any previous time, and these may include some as yet unidentified carcinogens. Some may be present in household products such as paints and cleaning agents, which often contain chemicals of unknown or dubious toxicity. Unidentified carcinogens possibly remain to be found in essential oils too. Increased exposure to chemical carcinogens does increase risk. Fire fighters, who are exposed to high levels of carcinogens such as benzene, formaldehyde, soot and styrene, have elevated levels of some cancers (LeMasters et al 2006). According to the WHO, 30% of all cancer deaths are caused by cigarette smoking, which exposes smokers to many chemical carcinogens. Certain populations may be overexposed to known environmental carcinogens such as aflatoxins, dioxins or pesticides. There are two basic types of carcinogen: those that react directly with DNA (DNA-reactive or genotoxic) and those that do not (epigenetic). Epigenetic carcinogens act in different ways, for example, by enhancing spontaneous mutation through stimulating cell proliferation, or increasing the amount of oxygen radicals in the cell (Williams 2001). They operate largely as promoters of cancer (‘promotion’ is explained below) and usually require high levels of sustained exposures. For example, dietary fat can act as an enhancer of cancer induction at about 40% of calories. The effects of epigenetic agents, unlike genotoxins, are reversible (Weisburger & Williams 2000). Some essential oil constituents have been found to exhibit estrogen-like activity in vitro or in vivo (see Chapter 11). This may be a concern for patients with estrogen-sensitive cancers, since there is a theoretical possibility of exacerbating tumor growth and proliferation. However, these estrogen-like actions in animals are mainly weak or limited to certain experimental conditions, and their affinity for estrogen receptors is generally extremely weak compared with that of 17β-estradiol. With the exception of (E)-anethole, we do not consider that essential oils containing these constituents pose a significant risk to patients with estrogen-sensitive cancers. The unregulated growth that characterizes most cancers is caused by damage to DNA, resulting in mutations to genes that encode for proteins controlling cell division. Many mutation events may be required to transform a normal cell into a malignant cell. Some chemicals, such as tobacco smoke carcinogens, directly form a bond with the chromosomal DNA of a cell, forming a DNA ‘adduct’, which can lead to gene mutations. For this reason they are described as being genotoxic. However, most genotoxic carcinogens, such as those found in foods and plants, require bioactivation to DNA-reactive products, usually via CYP enzymes (Weisburger & Williams 2000). Genotoxicity is a process that may or may not lead to cancer. In order for cell division and tissue growth to take place, chromosomal DNA must produce exact copies of itself. This process is not without errors, and mutations commonly occur. It is estimated that approximately 800 DNA bases per hour are damaged in the human body, due to mechanisms such as oxidation and methylation (Vilenchik & Knudson 2000). This ‘background’ level of DNA mutation can also be expressed as one lesion per million (106) bases. Adduct levels of less than one in 108 bases can be regarded as of no biological significance (Williams 2008). In healthy individuals, efficient DNA repair enzymes ensure that these defects do not lead to the growth of abnormal cells. Cut-and-paste repair mechanisms have been elucidated in some detail (Fortini et al 2003; Huffman et al 2005). In certain inherited genetic diseases, the activity of one of the DNA repair enzymes is reduced, and this can predispose an individual to some forms of cancer. DNA damage may be caused by highly reactive oxygen radicals (also known as reactive oxygen species, or ROS) and other intermediates of oxygen reduction. Oxygen radicals can attack DNA directly, or other cellular components such as lipids, leaving behind reactive species that in turn can bind with DNA bases (Valko et al 2004). Such oxidative processes, which may be either exogenous or endogenous in origin, can cause significant pre-carcinogenic changes. These include the mutation of oncogenes, tumor-suppressor genes and apoptosis-regulating genes, damage to mitochondrial DNA, and alteration of cell growth regulation pathways (Hursting et al 1999; Marnett 2000; Klaunig and Kamendulis 2004). The modification of gene expression by ROS has direct effects on cell proliferation and apoptosis.2 Apoptosis is a normal cellular process involving a genetically programmed series of events leading to the death of a cell. The involvement of ROS in the carcinogenic process highlights the importance of antioxidants as preventative agents, as well as the avoidance of pro-oxidants (Moller & Loft 2004). Essential oils with antioxidant properties are listed below, under Antioxidant activity.3 Although DNA damage can be measured in cells, this does not accurately predict the risk of cancer nor correlate with its extent, as this varies greatly with factors such as adduct type, frequency, persistence and ease of repair. Rather, it should be considered one of many factors in assessment (Jarabek et al 2009). For example, Table 12.1 shows the total of the two primary adducts detected for each test substance at three doses (Zhou et al 2007). Apart from high-dose dill apiole, the adduct levels for the apiole isomers are less than one in 108 bases, and so these molecules are very unlikely to be carcinogenic. The human hepatoma cells used here retain many of the phase I and phase II toxification and detoxification enzymes, but they have little or no CYP1A2, one of the most important enzymes for toxification of methyleugenol and estragole. One assay suggests that this enzyme is 53% more active in 1′-hydroxylating methyleugenol than estragole (Rietjens et al 2005a, 2005b). It is notable here that the formation of methyleugenol adducts is not dose related, neither is that for safrole at the high dose, and that the dose–response relationships for both apiole isomers have lower gradients than those for estragole and myristicin. Table 12.1 Relative adduct level (RAL) of some alkenylbenzenes in human hepatoma (HepG2) cells (Data from Zhou G-D, Moorthy B, Bi J et al 2007 DNA adducts from alloxyallylbenzene herb and spice constituents in cultured human (HepG2) cells. Environmental & Molecular Mutagenesis 48:715-721) Carcinogenesis is classically considered to evolve in three consecutive stages: initiation, promotion and progression. During initiation, the potential carcinogen bonds with cellular DNA, forming a DNA adduct. However, this step may have no adverse consequence if the cell efficiently repairs the DNA damage, or if it leads to cell death. In either case, the DNA adduct is short-lived, and will not lead to initiation. This is an important point, because a substance may appear to be mutagenic in an in vitro study, due to the appearance of transient DNA adducts (Rietjens et al 2005a). However if these are stable, the mutation will become ‘fixed’ in the cell, and then passed on to ‘initiated’ daughter cells. At this point the cell has been irreversibly altered, but is not yet malignant (Niesink et al 1996). Cancer promoters facilitate malignant growth by the selective, clonal expansion of initiated cells through an increase in cell proliferation. Promotion may involve altered gene expression enzyme activities, or the inhibition of DNA repair mechanisms. It is dose dependent and reversible upon removal of the tumor promotion stimulus. Progression, the third stage, involves processes such as abnormal cell growth, gene amplification (repeated copying of a segment of DNA), disruption of chromosome integrity, loss of tumor-suppressor activity, angiogenesis (the creation of a blood supply for a growing tumor), invasion and metastasis (Hursting et al 1999; Klaunig & Kamendulis 2004). A chemical that is only a promoter or an initiator is not a ‘complete carcinogen’. Initiated cells have to be subsequently and frequently exposed to doses of a promoter, over a certain period of time (which varies between chemicals) in order to induce malignant growth. A few essential oil constituents are clearly promoters but not initiators, as they only lead to neoplasia if administered subsequent to a known initiator. These include benzyl acetate, which promotes pancreatic tumors in rats, dodecyl acetate, α-phellandrene, and oxidation products of (+)-limonene, all of which promote skin tumors in mice [(+)-limonene itself is anticarcinogenic].4 Complete carcinogens are both initiators and promoters, and so are able to produce malignant changes by themselves.5 The alkenylbenzenes α-asarone, β-asarone, estragole, methyleugenol and safrole are all carcinogenic in rodents (see Constituent profiles, Chapter 14). However, before they can produce malignant change, these complete carcinogens go through a two-stage process of enzyme-activated biotransformation. Estragole, for example, is activated to the ‘proximate carcinogen’ 1′-hydroxyestragole, which is further activated to the ‘ultimate carcinogen’, 1′-sulfooxyestragole (1′-hydroxyestragole sulfate).6 The amount of ultimate carcinogen formed is dependent on the activity of the relevant metabolic enzymes. Activity can be increased by inducing enzyme synthesis, or decreased by inhibiting it. Factors that can affect this process of activation are discussed below under Carcinogenicity testing, and Genetic susceptibility. 1′-Hydroxyelemicin has shown weak hepatocarcinogenicity in one species of male mouse (Wiseman et al 1987), but elemicin has shown anticarcinogenic activity in vitro (Ikeda et al 1998) and is not regarded as a carcinogen. Elemicin, (E)-anethole and eugenol may manifest very weak carcinogenicity at high doses in rodents, but at low doses in humans there is negligible risk, because epoxidation is much less prevalent in humans, and because low doses are more easily detoxified in all species (Solheim & Scheline 1980; Wiseman et al 1987; Smith et al 2002). The three isothiocyanates found in essential oils (allyl, benzyl and phenylethyl) have all induced bladder cancer in male rats, but this is related to the irritancy of the compounds. It may also be gender- and species-specific since, in a two-year assay of allyl isothiocyanate, neither male or female mice, nor female rats, developed bladder tumors (Bechtel et al 1998). The isothiocyanates all show promise as therapeutic agents for lung cancer, as does phenylethyl isothiocyanate for colon cancer (see Constituent profiles, Chapter 14). A few chemical carcinogens are so powerful that even a single exposure to a dangerous level is likely to result in cancer. Although it is difficult to grade levels of risk, we do know that in most of the studies reported for the carcinogens listed in Table 12.2, daily exposure to high oral doses over many months is required to induce cancer in rodents (neonates excepted). One reason for this is that only 1 in 1,000 or less preneoplastic lesions in the liver lead to tumor formation, and the continued presence of a carcinogen is required to enable it to overcome host resistance factors (Williams et al 2005b). Table 12.2 Essential oil constituents for which carcinogenic activity has been reported aSpecies-specific carcinogens, some of which are also gender-specific and/or organ-specific cTumors found at 10, 12, 15, or 18 months of age dIn the instances where ‘total dose’ is given, the doses administered were not equal. Cells have elaborate mechanisms for avoiding mutation, including DNA repair enzymes, and pathways that either regulate cell growth or suppress oxidative stress (Dixon & Kopras 2004). Detoxifying and antioxidant enzymes include glutathione S-transferase, glutathione peroxidase, glutathione reductase, superoxide dismutase, quinone reductase and UDP-glucuronyltransferase. Eugenol induces UDP-glucuronyltransferase (Yokota et al 1988; Yokota & Yuasa 1990), various sulfur compounds induce quinone reductase (Fukao et al 2004; Munday & Munday 2001), as do (+)-limonene, perillyl alcohol and phenylethyl isothiocyanate (Gerhäuser et al 2003) and thyme oil induces superoxide dismutase (Youdim & Deans 1999). Essential oils that induce glutathione S-transferase are shown in Box 4.1.8 Melatonin inhibited safrole-DNA adducts in rat liver by 98.7%, possibly due to activation of protective enzymes such as glutathione peroxidase (Tan et al 1993). Glutathione is central to one of the most important protective enzyme systems. Glutathione transferases, for example, catalyze the conjugation and detoxification of electrophiles, which are usually generated in response to free radical damage to lipids and to DNA (Ketterer 1988). Associations have been shown between glutathione depletion and cancers of the breast, stomach, colon, lung and prostate (Cai et al 2001; Perera et al 2002; Acevedo et al 2003; Saygili et al 2003; Todorova et al 2003). Glutathione depletion is associated with critical illness and with many chronic conditions such as emphysema, alcoholism, Crohn’s disease, macular degeneration, Parkinsonism, Alzheimer’s disease, HIV infection and chronic fatigue syndrome. It is also associated with old age (Samiec et al 1998). Consequently people with chronic disease, and older people, may be more susceptible to chemical carcinogenesis. The importance of antioxidants in the context of cancer has already been elaborated. Essential oils that scavenge 1,1-diphenyl-2-picrylhydrazyl (DPPH) radicals are shown in Table 12.3. Antioxidant constituents include benzyl alcohol, carvacrol, 1,8-cineole, eugenol, (−)-menthol, (6R)-(+)-menthofuran, (−)-menthyl acetate, methyl salicylate, γ-terpinene, thymol and thymoquinone (see Constituent profiles, Chapter 14). Some unsaturated aldehydes, including (E)-cinnamaldehyde, citral, perillaldehyde and safranal, activate the antioxidant responsive element (ARE), which regulates important cellular antioxidants (Masutani et al 2009). Antioxidants are also protective in many other ways, unrelated to cancer. The use of antioxidant essential oils during chemotherapy or radiation is controversial. While it may protect normal cells from collateral damage, it may also protect the cancer cells that the treatment is designed to eliminate. Restriction on the use of essential oils would therefore seem appropriate (see final point of). There are a variety of antimutagenic factors (often antioxidants) that occur naturally in the diet such as vitamin E, β-carotene, D-glucaric acid, selenium, ascorbic acid and uric acid. Like glutathione, these act either by directly reacting with the mutagens or by suppressing cellular mutagenesis (Azizan & Blevins 1995). They can also act synergistically to prevent tumor initiation, promotion and progression (Hanausek et al 2003). Antioxidant and antimutagenic essential oils may therefore perform a valuable role in protecting against carcinogenesis. Essential oils that have demonstrated an antimutagenic action include those from blue chamomile (Hernandez-Ceruelos et al 2002), lavender (Evandri et al 2005), nut grass (Kilani et al 2005), rosemary (Fahim et al 1999), Dalmatian sage (Vukovi In addition to these effects, essential oils may act more directly, as anticarcinogens. This action is discussed below, under Chemoprevention. Several essential oils and essential oil constituents have been shown to stimulate immune responses. For example, eucalyptus oil induced morphological and functional activation of human monocyte-derived macrophages in vitro, dramatically stimulating their phagocytic response (Serafino et al 2008). Carvone and (+)-limonene increased the total number of white blood cells, and stimulated antibody production in spleen and bone marrow when administered to mice at 100 μmol/kg bw (Raphael & Kuttan 2003b). A feed additive containing the essential oil and dried plant parts of Origanum vulgare showed non-specific immune stimulant effects on porcine CD4 and CD8 immune cells, MHC class II antigen and non-T/non-B cells in peripheral blood lymphocytes compared to control animals (Walter & Bilkei 2004). For most of the 20th Century it was believed that the immune system was not able to protect against cancer development, but that view has now radically altered. In the 1980s evidence began accumulating that cells such as macrophages could kill cancer cells in vitro (Caignard et al 1985), but in vivo evidence was lacking until Shankaran et al (2001) demonstrated that lymphocytes and interferon-gamma (IFN-γ) collaborate to inhibit the development of carcinogen-induced sarcomas and spontaneous epithelial carcinomas, and that together they function as a tumor-suppressor system. We now know that cancer ‘immunosurveillance’ also involves, for example, other interferons, natural killer (NK) cells and T cells (Dunn et al 2004, 2006; Ghiringhelli et al 2006). NK cells act directly against tumor cells, and also secrete potent cytokines, such as IFN-γ (Miller 2001).7 There is evidence that immune stimulation is one mechanism through which essential oil constituents play a protective role. For example, benzaldehyde inhibited pulmonary metastasis in mice through an augmentation of NK cell activity (Ochiai et al 1986; Masuyama et al 1987, 1988). NK cell activity was enhanced in vitro by linalyl acetate (Standen et al 2006). A similar effect is reported for the essential oils of various trees native to Japan, including Thujopsis dolobrata stems and Chamaecyparis obtusa leaves, and for α-pinene, 1,8-cineole and (+)-limonene, all at concentrations of 0.05 or 0.1 ppm. This was partially mediated by induction of intracellular perforin, granzyme A and granulysin (Li et al 2006). The increased survival of mice with ascites tumors was associated with a restoration of NK cell activity to normal levels after four daily ip doses of β-caryophyllene at 20 mg/kg (Da Silva et al 2007). Eugenol dose-dependently enhanced mouse NK cell activity in vitro between 0.25 and 2.5 μmol/kg bw. It also showed a biphasic effect on plaque-forming antibody responses of mouse splenocytes to sheep erythrocytes in vivo, inhibiting these responses at about 0.1 μmol/kg bw, and enhancing them at about 0.25 μmol/kg. In vitro, eugenol was cytotoxic above 1.0 mM (Vishteh et al 1986). Benzyl alcohol had an inhibitory effect on cell proliferation and on induction of lymphokine-activated killer cell activity. Dose-dependent reductions in cell proliferation were seen when lymphocytes were incubated with 4 μL/mL of benzyl alcohol in heparin salt solution or in saline (Marincola et al 1987). It has been suggested that the anticarcinogenic activity of costus oil may be due to immune stimulation caused by the allergic skin response to the lactone content of the oil (Takanami et al 1987). The degree of dermal inflammation caused by costus oil correlated with the development stage of human breast, colon, lung and stomach cancers; the more virulent the reaction the higher the lymphocyte count, and the better the prognosis (Takanami et al 1983, 1984, 1985). Lymphocyte proliferation is stimulated by (+)-limonene in vitro (Manuele et al 2008), and in vivo immune enhancement has also been seen. There were marked increases in both the number and the phagocytic activity of alveolar macrophages in rats fed (+)-limonene for eight days (Hamada et al 2002). In lymphoma-bearing mice fed (+)-limonene for 9 days, macrophage phagocytosis was restored to normal levels, and nitric oxide production in macrophages was increased (Del Toro-Arreola et al 2005). Concurrent treatment with diallyl disulfide significantly inhibited the depression in leukocyte count and spleen weight experienced by rats receiving chemotherapy with fluorouracil (Sundaram & Milner 1996). Since immune competence is important in relation to cancer, it would be prudent to avoid essential oils that compromise the immune response in people with cancers (though they may be helpful in other clinical conditions). This especially applies to T cells in patients receiving chemotherapy. For example in mice, ginger oil inhibited T lymphocyte proliferation, and decreased the number of total lymphocytes and T helper cells (Zhou et al 2006). Studies using mouse splenocytes show in vitro inhibition of T lymphocyte proliferation by (E)-anethole, eugenol and isoeugenol (Yea et al 2006; Park et al 2007). Black seed essential oil reduced the antibody titer and splenocyte and neutrophil counts in rats, while increasing peripheral lymphocytes and monocytes (Islam et al 2004). The clinical relevance of these findings is not known, but they suggest that some essential oils might exacerbate lymphocyte depletion in patients receiving chemotherapy. Restriction on the use of essential oils would therefore seem appropriate (see final point of Summary). Essential oils may cause systemic allergic reactions through involvement of the immune system. For example, a 60-year-old man suffered a widespread rash and had an elevated white blood cell count after ingesting about 3 mL of tea tree oil, although he had taken it three or four times before without any undesirable effects except for itchy palms (Elliott 1993). The main predictive assays for carcinogenicity are mutagenicity/genotoxicity tests and live rodent tests. Generally, only a substance that tests positive in both areas is classed as a potential human carcinogen. However, in addition to genotoxic carcinogens, there are non-genotoxic carcinogens and genotoxic non-carcinogens (Sakai et al 2002). There are also species-specific and gender-specific carcinogens, which only lead to cancer in one species, or in one gender of one species. Types of molecular structure that give rise to suspicion of carcinogenicity are known as ‘structural alerts’. Alkenylbenzenes are clearly a structural alert in essential oils, since several of them are carcinogenic (Figure 12.1). However, some are not, and it can take years of testing before a single substance can be classified with confidence. In order to evaluate human risk, epidemiological data are helpful if available. No such data exist, however, for any essential oils or constituents, with two exceptions: furanocoumarins + UV light (see Ch. 5, p. 89), and safrole (see Betel quid: a case study below). When there are no epidemiological data, the comparative toxicokinetics of the chemical in rodents and humans may be studied. If there are no significant differences, this increases the likelihood that the rodent carcinogen is also a human carcinogen. Interspecies differences may mean the chemical is carcinogenic to rodents but not humans. Genetic mutations have already been described. They can be inherited across generations of cells, and may arise spontaneously or as a result of environmental factors. A genotoxic chemical can also be described as mutagenic. It has been postulated that mutagenesis plays a role in both the initiation and progression phases of carcinogenesis (Dixon & Kopras 2004). When an unfavorable DNA mutation occurs, repair mechanisms are engaged, and these have variable degrees of efficiency. Some carcinogens enhance mutagenesis through suppression of these mechanisms. In somatic cells, surviving mutations may lead to the development of cancer, while in fetuses they can cause effects such as malformations and spontaneous abortions. It is notable that the frequency of human chromosomal aberrations in spontaneous abortions within 12 weeks is 61.4%, compared to 0.65% in surviving neonates (Niesink et al 1996). α-Asarone has caused fetal malformations when given to pregnant mice (Salazar et al 1992); kidney and liver tumors were found in the offspring of female mice given four small doses of safrole (Vesselinovitch et al 1979). Many tests have been developed for detecting chemical mutagens. In vitro methods may employ bacteria, bacteriophages, human or other mammalian cells, while in vivo tests may be conducted in fruit flies or mammals. Tests for mutagenicity are carried out in non-mammalian cells or fruit flies, while tests for genotoxicity are carried out in mammalian cells, although the two terms are often used as if they were synonymous. Approximately 74% of carcinogenic chemicals are mutagenic in the Ames test, probably the best-known and most widely used assay. This is also known as the Salmonella/microsome assay or bacterial reversion assay, and was developed by Bruce Ames and others (Ames et al 1973; Mortelmans & Zeiger 2000). Various strains of the bacterium Salmonella typhimurium are used, each being sensitive to a different type of mutagenicity. Alkylating chemicals, such as epoxides, form the largest group of mutagenic agents. (An alkylating agent is a reactive chemical that can irreversibly alter the structure of another, such as DNA, by attaching an alkyl group.) Epoxides are not often found as constituents of essential oils, but may be formed during oxidation or through bioactivation (Table 12.4). Since these are inactivated almost as soon as they are activated, they do not have sufficient time to cause mutations. They are inactivated more efficiently in humans than rodents (Guenthner & Luo 2001). Table 12.4 The action of potentially toxic epoxide metabolites Although known carcinogens frequently test positive in genotoxicity assays, it has been recognized for over 20 years that there is a high proportion of false positives (Tennant et al 1987). Of 533 carcinogens, 93% were positive in at least one of three commonly used assays. However, 75–95% of non-carcinogens also tested positive in at least one assay, and this was not due to very high dosing. Surprisingly, tests using mammalian cells were more likely to give rise to false positives than the Ames test (Kirkland et al 2005). The high false-positive rate may be due to the failure of in vitro genotoxins to express their genetic toxicity in whole animals. Another possible factor is ‘genetic drift’ in rodent cell lines that have been cultured and sub-cultured innumerable times in multiple laboratories over decades (Tweats et al 2007). Despite these difficulties, a positive finding in all of three specific tests suggests that the substance is more than three times more likely to be a rodent carcinogen, and three negative results suggest it is more than twice as likely to be a non-carcinogen. The tests used were the Ames test, the mouse lymphoma assay, and either an in vitro micronucelus or chromosomal aberration test (Kirkland et al 2005). Several essential oils have tested positive in mutagenicity tests. For example, both dill weed and European dill seed oils induced CA in human lymphocytes and also were genotoxic in fruit flies (Lazutka et al 2001). The major constituent in both oils, (+)-carvone, has been reported as both genotoxic and non-genotoxic, but it is not carcinogenic, suggesting that neither dill seed nor dill weed oil is either. Scots pine needle oil was genotoxic in fruit flies and weakly induced CA in human lymphocytes (Lazutka et al 2001). The oil contains no known carcinogens. Peppermint oil was not mutagenic in the Ames test, but was marginally mutagenic in a CA test using Chinese hamster fibroblasts (Ishidate et al 1984). Lazutka et al (2001) reported that peppermint oil induced CA in human lymphocytes and also was genotoxic in fruit flies. Menthone (isomer not specified) has shown similar genotoxicity (Franzios et al 1997) and may be responsible for that of peppermint oil. However, it was not carcinogenic in mice (Stoner et al 1973). Peppermint oil was not mutagenic in a mouse lymphoma assay, and was not genotoxic in rat hepatocytes (Heck et al 1989). Some constituents have one or more reports of mutagenicity, but these are outweighed by other data that suggest they are non-carcinogenic. Examples include benzyl acetate, citronellal, linalyl acetate, isoeugenol, diallyl disulfide and diallyl sulfide. In other cases, such as piperonal, there are insufficient data to make a judgement. Low levels of genotoxicity have been reported for the alkenylbenzenes myristicin, parsley apiole and dill apiole, but rodent testing has so far failed to show any carcinogenicity for these constituents. (E)-Anethole, benzyl alcohol, (+)-carvone, (E)-cinnamaldehyde, eugenol, geranyl acetate and guaiacol are non-carcinogens with some false-positive genotoxicity assays (see Constituent profiles, Chapter 14). Thymol, carvacrol and γ-terpinene have demonstrated genotoxic activity in human lymphocytes in high concentrations, and antigenotoxic activity in low concentrations (Aydin et al 2005). All three compounds are notable antioxidants (see Constituent profiles, Chapter 14). Eugenol, another powerful antioxidant, has shown genotoxic, non-genotoxic and antigenotoxic properties in different tests, and is antioxidative in low doses and pro-oxidative in high doses (see Eugenol profile, Chapter 14). This apparent conflict is also seen in thymol, where a concentration of 0.2 mM was genotoxic to human lymphocytes when used on its own, but when combined with a mutagen at the same concentration was antigenotoxic (Aydin et al 2005). Both carvacrol and its isomer thymol prevented DNA strand breaks in human cells in vitro in the presence of the oxidant hydrogen peroxide (Slamenová et al 2007). Similarly, human colon and liver cancer cell lines treated with thymol or carvacrol at non-toxic doses showed significant resistance to DNA damage induced by hydrogen peroxide (Horváthová et al 2006). An Origanum compactum oil with 30.5% carvacrol and 27.5% thymol was genotoxic to mitochondrial DNA in Saccharomyces cerevisiae, but the absence of nuclear DNA damage suggests that long-term genotoxic risks are unlikely (Bakkali et al 2005). In some cases, reactive metabolites are removed by scavenging systems, such as glutathione, before they can bind with DNA. For example, there are some potentially mutagenic metabolites of essential oil constituents, such as estragole 2′,3′-oxide and eugenol 2′,3′-oxide, that are rapidly and efficiently detoxified (Table 12.4). This process, known as ‘inactivation’, prevents the formation of mutagens, so long as the scavenging systems are operating well, which they may not be in the very old or in people with chronic disease. In an in vitro study of substances used in dental practice, eugenol, guaiacol and thymol induced CA in human dental pulp cells after 30 hours exposure, but were not genotoxic after 3 hours (Someya et al 2008). Metabolites such as eugenol 2′,3′-oxide were thought to be responsible for this toxicity, which would probably not occur in vivo, since they would be rapidly inactivated. The short half-life of essential oil constituents in vivo is also likely to be a protective factor. Perhaps the most likely reason that the alkenylbenzenes eugenol, elemicin and (E)-anethole are positive in some tests for genotoxicity but are not carcinogenic, is that they only produce one mutation, one type of DNA adduct (in mouse liver) as do parsley apiole and dill apiole (Phillips et al 1984). However, at least two mutations are required to cause cancer, one in an oncogene and one in a tumor-suppressor gene, and in most cases a minimum of three or four mutations is needed before cells become malignant (Vogelstein & Kinzler 1993; Williams et al 2005b). Safrole, estragole and methyleugenol each produce four DNA adducts (Phillips et al 1984), though very low doses may only produce two adducts, as was seen in rats given 10 mg/kg of safrole orally (Daimon et al 1998). A substance that produces only one DNA adduct could stimulate repair mechanisms that have antigenotoxic effects. The manifestation of both genotoxic and antigenotoxic effects would then make sense, especially in potent antioxidant substances such as eugenol or thymol. Two aldehydes, cinnamaldehyde and vanillin, cause a type of mutagenicity that elicits recombinational repair, which fixes not only the damage caused by aldehyde, but also other DNA damage, resulting in both mutagenic and antimutagenic findings (Shaughnessy et al 2006; King et al 2007). Sekizawa & Shibamoto (1982) raised the issue of compound purity in mutagenesis testing. They found that estragole of 96% purity was weakly mutagenic in four Salmonella strains without S9, but 99.9% pure estragole was not mutagenic in these strains, with or without S9. They point out that compound purity for safrole has not always been specified. Photomutagens are by definition only mutagenic in the presence of UV light, and this is covered in Chapter 5. Some photomutagenic furanocoumarins are antigenotoxic or anticarcinogenic in the absence of UV light (Lee et al 2003; Takeuchi et al 2003). Linear furanocoumarins such as imperatorin and isopimpinellin had a greater inhibitory effect on DMBA-DNA adduct formation in mouse mammary glands compared to simple coumarins such as coumarin and limettin (Prince et al 2006). In a less commonly used test, infant mice are injected ip or sc with test substance either on day one of life, or in four escalating doses over the first three weeks. They are checked for tumor growth at 10–18 months. This protocol was used in all the α-asarone tests, all but one of the estragole tests, and some of the safrole tests (Table 12.2). Questions have been raised about using this method for carcinogens that require metabolic activation, since infants have immature hepatic enzyme function. However, test results suggest that it is a method with high sensitivity to weak carcinogens. After allowing for differences in route of administration and body weight, Epstein et al (1970) calculated that infant mice develop liver tumors at doses of injected safrole 1,100 × lower than the oral dose needed to produce similar tumors in adult mice. The production of pulmonary tumors by safrole in infant mice is notable, since safrole does not produce these in adult mice (Epstein et al 1970). Clearly, infant mice are highly susceptible to injected carcinogens. There are arguments to support the predictive value of rodent carcinogenicity testing. Most human carcinogens are also carcinogenic in more than one animal species. Experimental evidence of carcinogenicity has, in some cases, preceded human observation and there is a good correlation between animal and human target organ sites (Tomatis 1979). However, the relevance of the high doses used in many animal tests to actual human exposure has always been controversial (Zangouras et al 1981). ‘There is little sound scientific basis for this type of extrapolation, in part due to our lack of knowledge about mechanisms of cancer induction, and it is viewed with great unease by many epidemiologists and toxicologists. Nevertheless, to be prudent in regulatory policy, and in the absence of good human data (almost always the case), some reliance on animal cancer tests is unavoidable’ (Ames et al 1987). A significant problem in extrapolating animal data to humans is the relatively high occurrence of spontaneous tumors in laboratory animals. These tumors are caused, to a significant extent, by genetic factors and/or viruses that do not exist in the human species (Toth 2001). For DNA-reactive carcinogens in general, at least one rodent species is equally responsive or more responsive than the most sensitive human group (Williams et al 2005b). In a review comparing DNA repair activity in different species relative to that of rats (rat = 1), mice were 0.9, and humans 5.3, suggesting that human DNA repair activity is 5–6 times more efficient than that of mice or rats (Cortopassi & Wang 1996). In Europe, only data from rats (supported by other appropriate data) may now be used for the licensing of human medicines (Battershill & Fielder 1998). This means that live tests in mice for carcinogenicity are no longer regarded as useful (for pharmaceutical tests) by European regulatory authorities, although genetically bred mice may be used in future. The US has declined to support this position, and continues to test carcinogens in both species. Benzaldehyde, (+)-limonene and coumarin are epigenetic, species-specific, organic-specific carcinogens (citral probably is too), that do not present any carcinogenic risk to humans, and all have been used as anticancer drugs (see Constituent profiles, Chapter 14). In spite of this, they are often referred to as being carcinogenic, notably on websites warning of carcinogens in common foods. Interspecies differences in metabolism are even seen in probable human carcinogens such as safrole and estragole. When safrole was orally administered to both rats and humans, 1′-hydroxysafrole and 3′-hydroxyisosafrole were found as conjugates in rat urine, but not in human urine (Benedetti et al 1977). However, since safrole produces a multitude of metabolites, this does not necessarily mean it is not carcinogenic in humans. There is insufficient information to specify different safe exposure levels for different groups of individuals, although some are undoubtedly at greater risk than others. At-risk groups include infants, the very old, smokers, heavy drinkers, people with an occupational exposure to carcinogens, people with a personal or family history of cancer, and those with genetic susceptibilities to specific carcinogens. Wild & Kleinjans (2003) have reviewed the question of increased susceptibility in children. Genetic susceptibilities do exist for essential oil constituents, but we do not know who these individuals are. Pharmacogenetics was introduced on Ch. 4, p. 56, and is a new area of study attracting much interest. The capacity of individuals to metabolize a specific chemical can vary, because genetic differences can lead to the creation of different metabolizing enzymes. Such variations are known as polymorphisms and are often related to ethnic origin.8 In 13 human liver samples, the rate of methyleugenol 1′-hydroxylation varied by up to 27-fold, implying that methyleugenol toxicity is subject to wide variability in the population (Gardner et al 1997). Other research suggests a variation of only fivefold for methyleugenol, and CYP1A2 was identified as the most important enzyme for bioactivation to 1′-hydroxymethyleugenol, with CYP2C9, 2C19 and 2D6 playing lesser roles (Jeurissen et al 2006). A similar enzymatic profile has been elucidated for estragole, with CYP1A2 and 2A6 as the major players (Rietjens et al 2005a; Jeurissen et al 2007). In the case of safrole, CYP2A6, 2D6, 2C19 and 2E1 play a more or less equal role (Jeurissen et al 2004). Substances that inhibit these particular CYP enzymes, such as blue chamomile oil, may therefore afford some protection (Box 4.1). Estragole, methyleugenol and safrole compete for the catalytic sites of CYP1A2 and 2A6, the main enzymes responsible for bioactivation. 1′-Hydroxlation of equal concentrations of estragole and methyleugenol, for example, amounted to only 50% of the expected metabolism. Therefore a reduction of expected toxicity is likely when these constituents are both present in an essential oil (Jeurissen et al 2007). Genetic polymorphisms may result in either increased or decreased sensitivity to these carcinogens. Lifestyle differences such as alcohol use (in the case of safrole), or smoking and barbiturate use (for safrole, estragole and methyleugenol) could increase sensitivity because they may increase the activity of the relevant CYP enzymes (Jeurissen et al 2004, 2006; Rietjens et al 2005a). Differences in diet, or interactions with prescribed drugs such as ibuprofen may lead to different capacities for detoxification of 1′-hydroxyestragole (Iyer et al 2003). Variations such as these suggest that caution is needed in setting safe levels for human consumption. The metabolism of many chemicals is dose-dependent and this is of particular significance when biotransformation produces a carcinogenic metabolite. ‘At low doses a compound may be safely disposed of metabolically whereas at higher doses these pathways become saturated and alternative routes become increasingly employed which lead to the formation of toxic metabolites’ (Zangouras et al 1981). Hepatotoxicity is seen, for example, with (E)-anethole in high doses. In female rats chronic hepatotoxicity and a low incidence of liver tumors were evident at a dietary intake of 550 mg/kg/day. At this dose, daily hepatic production of anethole 1′,2′-epoxide (AE) exceeded 120 mg/kg body weight. However, there was no hepatotoxicity when the daily hepatic production of AE was below 30 mg/kg (Newberne et al 1999). Methyleugenol, estragole, α-asarone, β-asarone and safrole cause liver tumors in rodents after repeated dosing, and two key factors contribute to tumor growth. Firstly, the degree of oxidative metabolism is increased due to the dose-related induction of a CYP enzyme. Secondly, dose-dependent hepatotoxicity occurs which seems to be a necessary precursor to cancer in the liver. At low doses, these factors are much diminished. CYP induction has not been observed in rats receiving oral doses of less than 10 mg/kg of estragole, methyleugenol or safrole for five days, and the formation of toxic 1′-hydroxy derivatives is greatly reduced. The dose of methyleugenol needed to cause hepatotoxicity in rodents is estimated to be in the range of 1–10 mg/kg/day (Smith et al 2002). The formation of estragole or methyleugenol-DNA adducts depends on activation to the 1′-hydroxy metabolite, and on how much of it is formed (Gardner et al 1995). Adduct levels of at least 15 pmol/mg of DNA at 23 days are required for statistically significant tumor formation (Phillips et al 1984). At low doses, it is presumed that any reactive species formed from 1′-hydroxy derivatives are effectively inactivated by glutathione and other compounds. This is evidenced by the transience of adducts formed after low doses. A rapid drop in adduct formation occurred within 7 days following the ip administration of a 2 or 10 mg dose of estragole, methyleugenol or safrole (equivalent to 100 or 500 mg/kg) to mice (Randerath et al 1984). In mice given 12 μmol of [2′,3′-3H]1′-hydroxyestragole ip, the three adducts of estragole-deoxyribonucleoside were removed rapidly from mouse liver DNA (Phillips et al 1981a). The percentage of 1′-hydroxyestragole excreted in urine after oral doses of estragole increased from 1.3% to 13.7% in rats, and from 1.3% to 9.4% in mice as the dose was increased from 0.05 mg/kg to 1,000 mg/kg (Anthony et al 1987). In humans, the amount of 1′-hydroxyestragole found in excreted urine was only 0.3% following ingestion of 100 μg of estragole (Sangster et al 1987). Lower doses of estragole and methyleugenol are detoxified much more efficiently than higher doses, preventing hepatotoxicity and adduct formation. If detoxification is more efficient in humans than rodents, this should be factored into safety considerations. In a recent review of physiologically based biokinetic models for estragole, methyleugenol and safrole, it was concluded that in spite of differences in the rate of specific metabolic conversions, the overall bioactivation levels for all three are comparable, which is in line with their comparable carcinogenic potential (Martati et al 2011). The idea that some carcinogens are more potent than others was first mooted in 1939 and eventually led, in 1984, to the proposal of a TD50 for carcinogens; the criterion being the appearance of malignant tumors in 50% of animals within a fixed period (Sawyer et al 1984). The TD50 is a useful measure of the relative carcinogenicity of substances, and takes data from many rodent studies. The information in Table 12.5 suggests that, in mice, estragole and safrole are equally potent, and methyleugenol is 2.6 times more potent than either. In rats, benzo[a]pyrene is almost 500 times more potent than safrole. While the TD50 does not incorporate the notion of a threshold per se, it naturally gives rise to it. In 1990, the HADD (highest average daily dose) was proposed for carcinogens by an EPA researcher, on behalf of the CIPEMC (Nesnow 1990). The HADD is the highest dose administered in a cancer study that did not give rise to a statistical increase in tumors, and is analogous to a NOAEL. Table 12.5 Comparison of carcinogenic potencies in rodent studies (Data from The Carcinogenic Potency Project [http://potency.berkeley.edu/index.html accessed April 28th 2012]). The concept of hormesis was well established in toxicology 100 years ago, it fell out of favor in the 1930s, and has only re-emerged as a credible model since the 1980s (Calabrese 2002). Proponents of hormesis believe that it is erroneous to assume that the LNT model applies universally to carcinogens (Calabrese & Baldwin 2003). Hormesis applies to any situation in which a carcinogenic substance has completely different, often opposite, effects at different doses. Hormesis is more widely accepted in relation to epigenetic carcinogens than genotoxic ones. Evidence of hormesis was identified in 350 of 4,000 potentially relevant articles scanned by Calabrese & Baldwin (1998). The finding of opposite effects at different doses is not uncommon in pharmacology, and is often referred to as ‘biphasic’. A separate, and more controversial challenge to the LNT model for carcinogens has come from proponents of the threshold model. Gaddum (1945) pointed out that dose needs to be plotted on a logarithmic scale to achieve a linear response. A linear (arithmetic) scale for the dose of a chemical obscures effects at doses below those used in the experiment, and distorts the effects seen over the range of doses used (Waddell 2003). Waddell contends that conventional approaches to carcinogen dose–response relationships have concealed the presence of thresholds in major studies, and that the ‘Rozman scale’ should be used, since it is continuous down to one molecule, and so permits the plotting of very small doses (Rozman et al 1996; Waddell et al 2004).9 Research by others supports this idea. Williams et al (2005a) reported that very low levels of two carcinogens were not hepatocarcinogenic in rats, and that the appearance of DNA adducts was non-linear in respect to dose. They therefore cautioned against extrapolation from high-dose effects to potential carcinogenicity at low doses. As an example of the Rozman scale, data from the NTP study on methyleugenol (National Toxicology Program 2000) and DNA adduct data (Carmichael et al 1999), shows a linear response for methyleugenol, and indicates that tumor formation closely follows adduct formation (Figure 12.2). The threshold dose for adduct formation in rats was 1020.07 molecules of methyleugenol/kg/day.10 This is equivalent to 34.8 mg/kg/day, which is about 10 times less than the dose that produced tumors in rats (Waddell et al 2004). Using data from carcinogenesis assays, linear responses have also been found for allyl isothiocyanate, estragole and benzyl acetate, with thresholds of 4.14, 174 and 321 mg/kg/day, respectively. When compared to estimated levels of human daily exposure, these results suggest that considerable margins of safety exist for all these constituents.
Cancer and the immune system
Cancer and carcinogens
DNA damage
Initiation, promotion and progression
Carcinogenic essential oil constituents
Defense mechanisms
Induction of detoxifying enzymes
Antioxidant activity
Antimutagenesis
-Ga
i
et al 2006), and turmeric rhizome (Jayaprakasha et al 2002). Many essential oil constituents are also antimutagenic. Perillyl alcohol, for example, effectively blocks the binding of the carcinogen DMBA with DNA, probably via enzyme inhibition (Chan et al 2006).
The immune system
Immunity and cancer
Possible adverse effects
Carcinogenicity testing
Mutagenicity/genotoxicity tests
Constituent
Epoxide metabolite
Action of metabolite
(E)-anethole
Anethole 1′,2′-oxide
Possibly mutagenic, carcinogenic in rats, less is formed in humans, detoxified at low doses
α-Asarone
Asarone 1′,2′-oxide
Mutagenic, carcinogenic
Coumarin
Coumarin 3,4-oxide
Hepatotoxic in rats, much less is formed in humans
Estragole
Estragole 2′,3′-oxide
Mutagenic but not carcinogenic, rapidly detoxified, especially in human liver
Eugenol
Eugenol 2′,3′-oxide
Potentially mutagenic, rapidly detoxified, especially in human liver
Safrole
Safrole 2′,3′-oxide
Potentially mutagenic, rapidly detoxified
Essential oils and their constituents
Live animal tests
Interspecies differences
Risk factors
At-risk groups
Genetic susceptibility
Exposure
Detoxification and dosage
Thresholds
Constituent
Rat TD50
Mouse TD50
Allyl isothiocyanate
96
No positive test results
Benzaldehyde
No positive test results
1,490
Benzo[a]pyrene
0.9
3.5
Benzyl acetate
No positive test results
1,440
Coumarin
39.2
103
Estragole
No tests done
51.8
(+)-Limonene
204
No positive test results
Methyleugenol
19.7
19.3
Safrole
441
51.3
Stay updated, free articles. Join our Telegram channel
Full access? Get Clinical Tree
Cancer and the immune system
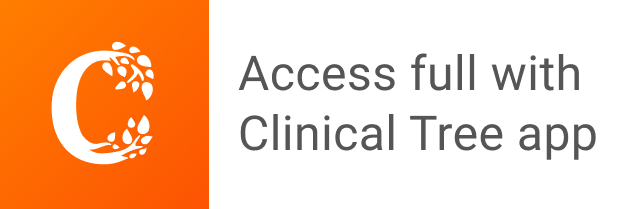