Fig. 10.1
The photomicrographs demonstrate immunohistochemical staining of 11beta-hydroxysteroid dehydrogenase type 1 (HSD1) activity in bone. HSD1 is an enzyme that interconverts inactive to active steroids. Samples were made up of sections of decalcified distal rat femur. HSD1 antibody was used to detect HSD1 enzyme. Immunopositive stain is shown by the brown colour staining
Abused Substance
Substance of abuse has been known to affect human body both psychologically and physiologically. The drugs which are commonly abused can be categorised according to several groups: stimulants (cocaine, amphetamine, nicotine), opioids and depressants (alcohol, benzodiazepines) and miscellaneous (marijuana, ketamine). These substances of abuse have been associated with many negative effects on various organs in the body [16]. The use of these substances may also affect the bone which is often overlooked and ignored. In this section, the effects of three substance of abuse on bone markers were discussed.
Nicotine
Nicotine , the major addictive substance present in cigarette, has been implicated in many conditions such as cancers, cardiovascular diseases, lung diseases, pregnancy-related complications, negative effects on children and many other conditions [17, 18]. Several reports have been published with regard to the effects of nicotine on bone. It was noted that at low dose, nicotine exerted stimulatory effects on bone. However, high dose of nicotine was shown to negatively affect bone in both in vivo and in vitro studies [19].
In terms of bone mineral density, there were conflicting results. In a study, female rats were exposed to cigarette smoke for the duration of either 2, 3 or 4 months. It was observed that bone mineral density was reduced in rats exposed to 4 months of cigarette smoke [20]. In another study using female rats, it was shown that neither low dose (0.4 mg/kg/day) nor high dose of nicotine (6.0 mg/kg/day) given for 1 year in drinking water had any significant effects on bone mineral density [21]. The different route of administration and duration of exposure to nicotine may have affected bone turnover differently.
Despite the inconclusive findings on bone mineral density, several other studies were consistent in reporting the adverse effects of nicotine on bone markers. In an in vitro study on human mesenchymal stem cells, nicotine caused a dose-dependent reduction effect on cellular proliferation and expression of bone morphogenetic protein-2 (BMP-2), which is a marker for bone formation [22]. Similar findings on BMP-2 were observed in rabbits which were implanted with nicotine pellet subcutaneously for 7 weeks [23].
Bone formation marker (bone-specific alkaline phosphatase) was found to be reduced, while bone resorption marker (tartrate-resistant acid phosphatase 5b) was found to be increased in rats exposed to cigarette smoke for 3 or 4 months [20]. In another study, nicotine given intraperitoneally at the dose of 7 mg/kg to male rats for 2 months was shown to increase the levels of bone-resorbing cytokines (interleukin-1 and interleukin-6) and bone resorption marker (pyridinoline), while causing a decrease in osteocalcin (bone formation marker). It was also shown that cessation of nicotine for 2 months did not reverse the negative effects of nicotine on these bone biomarkers [17]. However, postmenopausal women who smoked at least ten cigarettes per day had higher bone mineral density after cessation of smoking for 1 year compared to women who continued smoking. Cessation of smoking was associated with increased alkaline phosphatase levels [24].
Besides the bone metabolism markers, nicotine was observed to negatively affect bone histomorphometry parameters [25]. Nicotine at the dose of 7 mg/kg given intraperitoneally for 2 months caused a decrease in bone volume, osteoblast surface, double-labelled surface, mineral appositional rate and bone formation rate. Nicotine was also found to decrease trabecular thickness and increased osteoclast surface and eroded surface [26].
Nicotine was also proven to delay bone healing. A study was carried out to evaluate the effects of nicotine on tendon-to-bone healing in rats. Proliferating cell nuclear antigen immunohistochemistry analysis found that the cellular proliferation in the nicotine group was decreased. It was also shown that nicotine caused prolonged inflammation as evidenced by the presence of inflammatory cells. These effects led to delayed healing process and thus reduction in biomechanical strength of the bone [27]. The presence of tumour necrosis factor-α (TNF-α) was necessary to accelerate bone fracture healing and nicotine was found to inhibit the secretion of TNF-α, which may partly be responsible for causing the delay in healing [28].
Based on the above findings, it is undeniable that nicotine can cause detrimental effects on bone. However, nicotine may not be the sole culprit in delaying fracture healing. Other chemicals beside nicotine which are present in the cigarette smoke may also be responsible for these detrimental effects on bone [29].
Opioids
Opioids are a family of agents that are commonly used to relieve pain and are also often abused for its euphoric effects. Usage of opioid for long-term pain management is on the rise and concerns on its safety have been raised. The adverse effects of opioids on multiple organs may occur via various mechanisms [30]. Furthermore, long-term use of opioids may lead to endocrinopathy such as opioid-induced androgen deficiency [31] and its systemic effects including osteoporosis and osteopenia [32].
Prolonged heroin addicts were observed to have osteopenia at the femoral neck and distal forearm. These subjects also had increase bone turnover markers which were restored to normal levels after 1 year of methadone maintenance therapy [33]. Even though it seems that methadone maintenance therapy was able to reverse the effects of heroin addiction on bone turnover, long-term methadone therapy may also exert negative effects on bone. In a study population which has been on methadone maintenance therapy for a median of 11 years, it was found that the male subjects had lower bone mineral density as compared to control. However, this effect was not seen in female subjects [34]. The lack of effects on bone mineral density in women was also observed in another study involving perimenopausal women [35].
In another study which involved patients with heroin and buprenorphine addiction during the period of abstinence, it was found that ossalgia (bone pain) was one of the symptoms most prominently felt. It was also observed that the patients exhibited high clearance of calcium in the urine which was suggestive of high bone resorption [36].
Opioids have also been implicated in increasing fracture risk [37]. In a case-control study, it was found that risk of fracture was evident with acute use but not chronic use of opioid, which suggested the key role of acute central nervous system effects of opioids in fracture risk rather than the chronic opioid-induced hypogonadism [38]. As mentioned earlier, opioids have endocrinopathy effects, which may account for the adverse effects of opioids on bone. However, in one study, bone mineral density of the population studied showed lower bone mineral density regardless of their total testosterone blood levels [39].
In another perspective study, it was reported that the effects of opioids on bone may be attributed to the presence of opioid receptors on osteoblast cells. This was proven via reverse transcriptase polymerase chain reaction (RT-PCR) and immunohistochemistry analysis which showed the presence of three types of opioid receptors. It was also found that osteocalcin synthesis was inhibited by high concentrations of the mu agonists morphine (D-Ala(2), N-MePhe(4), Gly(5)-ol)-enkephalin) but not with the delta agonist (D-Ala(2), D-leu(5))-enkephalin). These effects were abolished when osteoblast cells were incubated simultaneously with naloxone, an opioid antagonist [40]. The existence of these receptors and the varying affinity of individual opioids towards these receptors may account for the different effects of these opioids on the bone. Amongst the opioids commonly used, tramadol was observed to have the least effects on bone as compared to morphine and fentanyl as evidenced by the biochemical and histological parameters [41].
Alcohol
Besides the two substance of abuse discussed above, chronic alcohol abuse is established as an independent risk factor for osteoporosis and has many effects on bone mass, bone metabolism and bone strength [42].
There is a correlation between chronic alcohol abuse and low bone mass which was prominent in males [43]. Alcohol consumption was found to decrease fat mass and lean mass which are directly related to bone mineral density [44]. Alcohol was also able to reduce serum estradiol level via the increase in receptor activator of nuclear factor κB ligand (RANKL) expression, which eventually led to decrease in bone mineral density [45, 46]. Conflicting bone mineral density findings were observed in another study. Moderate alcohol consumption in postmenopausal women showed an increase in bone mineral density, which was associated with reduction in biochemical markers of bone turnover [47]. This suggests that the inhibitory effects of alcohol on the bone turnover attenuate the imbalance in bone turnover associated with menopause.
Alcohol was shown to exert direct effects on bone by reducing osteoblast activity and differentiation as well as osteoblastogenesis, which resulted in decrease bone formation [48]. In addition, alcohol increased bone resorption by increasing osteoclastogenesis and osteoclast activity which was mediated by cytokines such as interleukin-6 (IL-6) and RANKL [49, 50].
Several studies have shown that alcohol intake in both human and rats resulted in reduced bone formation [51, 52]. Elevated blood alcohol concentrations for continuous periods of time during repeated binge cycles in male rats were found to alter bone remodelling [43, 53, 54]. Consequently, there were reduced bone formation and increased resorption as a result of remodelling imbalance. Studies in young and adult rats have found that alcohol can affect osteogenesis, leading to altered cortical and cancellous architecture, decreased bone mineral density and significant reduction bone strength [55–57]. The increased blood alcohol concentrations due to binge alcohol administration in male rats were responsible for alcohol-induced osteoporosis mediated by bone formation inhibition and bone resorption stimulation [58].
Alcoholics were also associated with risk of fractures [59]. Exposure to alcohol delayed fracture healing by disrupting the canonical Wnt signalling which plays a major role in fracture repair [60]. Exposure to different type of alcohol may affect the outcome of the fracture risk. Women who preferred wine had lower hip fracture risk compared to the other alcohol drinkers [61].
Phosphoric Acid in Carbonated Drinks
Humans consume approximately 1 500 mg of phosphorus daily from unprocessed foods, such as milk, cheese, beef, poultry, fish, etc. [62]. Consumption of phosphorus is further increase to more than double in the past few decades with increasing popularity of carbonated drinks and processed foods. Consumption of carbonated beverages continue to increase and is currently the preferred beverage for young adults worldwide [63]. Carbonated beverages contain phosphoric acid. The addition of phosphates in food processing has now become common practice, and this has also added to the intake of phosphate in humans [64]. The intake of phosphorus and phosphoric acid has been hypothesised to cause bone loss in humans. Although concrete association between high phosphorus intake and bone loss in humans is still being debated [65–67], various rat models for research have since been used to illicit this association.
Since the early 1970s, various studies have demonstrated that an increase in phosphorus intake would cause an increase in bone loss [63, 68–70]. This is especially true if the ratio of phosphorus to calcium intake was more than two times higher. Increasing the calcium intake may diminish the calcium loss. However, continuing increase of both phosphorus and calcium even with a ratio of 1:1 resulted in bone loss, indicating that the compensation of increased calcium intake to reduce bone loss due to phosphorus has its limitation [70, 71]. Draper et al. [71] demonstrated that osteoporosis can be induced in aging rats by high phosphorus diets. Calcium loss from skeleton, measured by calcium excretion, was increased by providing more than 0.3 % of phosphorus in the diet. Rats fed 0.6 %, 1.2 % or 1.8 % phosphorus diet had an average loss of 17.6 %, 40.2 % and 34.2 % of calcium, respectively, from bone when compared with rats fed with 0.3 % phosphorus. Garcia-Contreras et al. [63] further elicited that rats given cola soft drink had significantly lower femoral BMD when compared with rats given water. Histological femoral cortical width was also significantly lower amongst rats given cola soft drink.
There are a few postulated mechanisms regarding bone loss due to increase phosphorus intake in rats. Draper et al. [69, 71, 72], in a series of three papers, postulated that high phosphorus intake will result in secondary hyperparathyroidism, which leads to increase in bone resorption. This hypothesis was strengthened with the demonstration that bone loss does not occur in parathyroidectomised rats [68]. Later, it was found that phosphorus intake would inhibit renal 1α, 25-dihydroxyvitamin-D synthesis which blocks intestinal absorption and renal tubular reabsorption of calcium [73]. Increase phosphorus intake will therefore result in hypocalcaemia, secondary hyperparathyroidism and reduction in 1α, 25-dihydroxyvitamin-D level.
High phosphorus diet induces osteoporosis in rats. It can be safely assumed that with rats fed on high phosphorus diet, immunohistochemistry changes consistent with osteoporosis should be seen.
Caffeine
There were conflicting evidences on the association of caffeine intake with bone loss and increase risk of fracture in humans [74]. Several observational studies suggested a reduction in bone mineral density (BMD) with caffeine intake [75–77], while many others suggested no association [78–82]. It can be concluded that caffeine intake may have an effect on bone loss or calcium loss, but this effect can be easily offset by optimal intake of calcium according to recommended daily intake [74].
The effect of caffeine on bone structure yields similar conflicting results. Glajchen et al. [83] reported no effect on bone remodelling in adult rats, even at very high levels of caffeine consumption daily for 8 weeks. Sakamoto et al. [84] also concluded no effect of bone metabolism in adult rats given high coffee diets. However, a recent study by Liu et al. [85] demonstrated that caffeine enhances COX-2/PGE2-regulated RANKL-mediated osteoclastogenesis in cell culture model. Three-week-old rats fed with caffeine for 20 weeks demonstrated significantly lower bone mineral density of lumbar vertebra, femur and tibia compared with the control group. Calcium contents were also lower in the tibia and femur bones of the rats [85].
It is possible that caffeine intake in rats may cause bone loss changes in rat bones. However, the evidence is currently inconclusive and more research in this area is needed.
Metal Toxicity
Iron
Iron overload is a condition where there is accumulation of iron in the body. The most important cause of iron overload is a disease called hemochromatosis . In this disease, the body tends to absorb high amounts of iron from the diet and the excess iron is stored in organs, especially the liver, heart and pancreas.
Another condition which can lead to iron overload is by repeated blood transfusion. Each blood transfusion increases the iron level in the body. As the red cells break down over time, the iron in the haemoglobin is released. However, the body has no natural way to remove the excess iron. Therefore, a protein called transferrin carries iron for storage in various organs including the bone marrow. As the bone marrow is in close vicinity to the bone and the bone cells are derived from the progenitor cells in bone marrow, the excess iron could cause bone toxicity. This may lead to bone loss, which is reflected by changes in certain bone markers.
Recent human studies have shown that iron overload may be toxic to bone. In a study by Kim et al. [86], the iron level was measured in relation to changes in bone mineral density of several bone sites. It was proven that iron stores could be an independent risk factor for accelerated bone loss, even in healthy populations. In another study, the relationships between iron levels and bone metabolism in patients with hip fragility fractures were examined [87]. There was a high prevalence of iron overload in postmenopausal women with fragility fracture . Iron overload may lower bone mineral density and cause bone loss by enhancing the activity of bone turnover in postmenopausal women. Another proposed mechanism is that iron generates free radicals, which are toxic to bone cells. The toxic effects of iron could be assessed by measuring the bone turnover markers such as serum osteocalcin and C-terminal telopeptide (CTX) or other markers such as the bone-resorbing cytokines.
The toxic effects of iron overload on bone have been investigated in rodent models. One of the widely accepted iron overload models is the ferric-nitrilotriacetate (FeNTA) model . In this model FeNTA, a compound made up of ferum chelated with nitrilotriacetate, is injected intraperitoneally into rats. Ferum or iron is a strong oxidant, while nitrilotriacetate stabilises the compound. It is the iron component of FeNTA which generates free radicals through Fenton reaction [88]. Studies have shown that free radicals were toxic to bone and may cause a reduction in bone mineral density [89]. Sontakke et al. [90] have reported that subjects with high bone loss have elevated malondialdehyde level, an index of lipid peroxidation, while the antioxidant enzymes were reduced. In another study, Maggio et al. [91] showed that elderly women suffering from bone loss have low levels of natural antioxidants. Therefore, free radicals may be responsible for the pathogenesis of osteoporosis.
Several studies using the FeNTA rat model have demonstrated the toxic effects of iron overload and free radicals on bone. Iron was found to be deposited in bone cells and there was an increase in the number of osteoclast, the cells responsible for bone resorption [92]. Other negative effects of FeNTA include deterioration of the structural, static and dynamic parameters of bone histomorphometry [93], impairment of bone mineralisation [94] and decreased bone calcium [95]. In terms of the bone markers, FeNTA was shown to increase the levels of bone-resorbing cytokines, interleukin-1 and interleukin-6 accompanied by elevated levels of deoxypyridinoline cross-link, a bone marker of osteoclast activity [96].
Isomura et al. [97] have investigated the relationship between free radicals induced by iron and bone metabolism in young and postmenopausal female rats. This was achieved by giving dietary iron overload to rats and measuring several bone metabolic, oxidative stress and antioxidant markers. The markers were measured using enzyme-linked immunosorbent assay (ELISA ) technique, automated analyser or enzyme assay. Postmenopausal rats exhibited reduction in both the bone formation markers of serum alkaline phosphatase activity and osteocalcin level. However, there was no difference in the urinary excretion of deoxypyridinoline (DPD), a bone resorption marker. Iron-rich diet in postmenopausal rats has led to high excretion of DPD and 8-hydroxy-2′-deoxyguanosine, a marker of oxidative stress.
The iron-rich diet induced significant increases of serum osteopontin and tumour growth factor (TGF)-β1, resulting in augmented receptor activator of nuclear factor kappa B ligand (RANKL)-induced osteoclast formation [98–100].
TGF-β1 showed a negative correlation with serum glutathione peroxidase (GPx) activity, but a positive correlation with the serum iron level. The study has clearly shown that free radicals were toxic to bone and may result in osteoporosis, in which the concentrations of biochemical markers for oxidative stress and bone resorption were correlated to each other.
The opinion that bone toxicity of iron overload may be contributed by free radicals was supported by findings that antioxidants may reverse the toxicity. Vitamin E is an example of a lipid-soluble antioxidant which could suppress lipid peroxidation by breaking the peroxyl radical chain reaction. Vitamin E in the form of alpha-tocopherol was shown by Ebina et al. [93] to protect bone from FeNTA toxicity, while Yee and Ima Nirwana [95] had shown that palm oil-derived vitamin E could do the same. Palm vitamin E was shown to suppress interleukin (IL)-1 elevation and bone turnover marker changes induced by FeNTA [96].
Cadmium
Cadmium , a by-product of mining and smelting industry, is a highly toxic metal which exerts disruptive effects to the body at doses much lower than most other toxic elements. It is mainly used in the production of alloys and rechargeable batteries. In polluted areas, cadmium is released into rivers and remains in soil for several decades, taken up by plants and contaminates the food chains. It is also present in tobacco smoke. Acute and chronic cadmium intoxications can occur by inhalation and ingestion. Absorbed cadmium irreversibly accumulates in organs such as kidneys, lungs, the liver and bones [101, 102].
The “Itai-itai” (which literally translates to “it hurts-it hurts”) disease was the world’s first documented mass cadmium poisoning that took place in Toyama Prefecture in Japan in 1912. A mining company in the industrialised Toyama Prefecture released cadmium into the local river and poisoned everyone who drank the water or ate foods from the plants which were watered with the toxic metal. The first symptoms of the disease appeared as spinal and leg pain, later progressed to skeletal deformities, bone fractures even from minimal trauma, anaemia and kidney problems [103].
The toxic effects of cadmium on bone during the outbreak of Itai-itai disease led to many laboratory and epidemiological studies. Recent findings suggest that even relatively low chronic exposure to this metal may pose a risk for bone diseases. Increased prevalence of osteopenia and osteoporosis as well as fractures has been noted at low to moderate environmental exposure to cadmium [104, 105]. A study involving Chinese farmers who were exposed to cadmium from contaminated rice from over 20 years showed that these farmers had decreased bone mineral density [106]. Staessen et al. [107] reported that in subjects with high cadmium level in their urine, the risk for bone fracture was higher. Studies in laboratory animals also showed similar results. In rats treated with cadmium-infused drinking water, the BMD and skeletal mineral contents were lower than those of control rats. The cadmium-induced skeletal changes resulted in the weakening of bone biomechanical properties [108].
The detrimental effects of cadmium on bone occur through direct bone damage and indirectly due to renal dysfunction, which begins as renal tubular proteinuria, calciuria and phosphaturia. The disturbances in calcium and phosphate homeostasis that accompany cadmium nephropathy may lead to bone demineralisation and fractures [101]. In culture systems, cadmium acts directly on bone cells to retard bone formation and increase bone resorption. In a mouse osteoblast-like cell line, cadmium exposure led to decreased cellular alkaline phosphatase [109] and increased prostaglandin E2 secretion; the latter can stimulate formation and activation of osteoclasts, leading to uncoupling of bone remodelling [110]. As for bone-resorbing ability, cadmium has been shown to increase the number and activity of multinucleated osteoclast-like cells formed by the fusion of mononuclear precursors in primary rat osteoclast cultures [111]. Urinary markers for bone demineralisation were assessed in a study involving a group of school children exposed to cadmium in Pakistan. In these children, those with high urinary cadmium were noted to have high urinary calcium and deoxypyridinoline, suggesting that cadmium exposure is associated with bone resorption [112].
Lead
Lead is a poisonous metal which occurs both in organic and inorganic forms in the environment [113]. It is present in the air, water, soil, food and in many household products including brass plumbing fixtures, paints and cooking and eating utensils [114]. Routes of exposure mainly occur through the respiratory and gastrointestinal systems. Lead is conjugated in the liver and excreted through the kidneys, but most of the absorbed lead accumulates in various organs particularly bones, lungs, heart, brain and testes.
Toxicity to lead occurs where the levels in the blood circulation are high. Lead poisoning can take place acutely, but chronic poisoning as a result of continuous exposure is more common, affecting many bodily functions at the molecular, cellular and intercellular levels [115]. Lead intoxication induces oxidative stress by increasing lipid peroxidation and decreasing the antioxidant system. The endogenous antioxidant enzymes such as superoxide dismutase, glutathione peroxidase, glutathione S-transferase and catalase enzyme levels were noted to be lowered. In addition, lead toxicity also resulted in increased bilirubin and the enzymatic activity of glutamic-oxaloacetic transaminase, glutamate pyruvate transaminase and alkaline phosphatase [116–118].
Since bone is the major reservoir for body lead, the latter has profound detrimental effects to bone. Elevated lead in the body produces a characteristic dense metaphyseal lines on X-ray. In children, it has been associated with decreased stature and chest circumference [119, 120], along with reduced somatic growth, longitudinal bone growth and bone strength [121]. Lead exposure may lead to reduced bone density [122], osteopenia [123] and impaired bone mineralisation [124].
In vivo and in vitro studies showed that lead affects the bone by direct action on bone cell functions and indirectly via the changes in the circulating levels of the hormones and cytokines which modulate bone cells, particularly 1, 25-dihydroxyvitamin D3. High levels of lead in blood were associated with low level of 1, 25-dihydroxyvitamin D3 [125]. Lead induces chondrogenesis by altering multiple signalling pathways which include TGF-β, BMP, AP-1 and NFκB [126]. Lead adversely affects bone formation and resorption by targeting cellular pathways involving osteoblasts and osteoclasts. It significantly reduced the activity of alkaline phosphatase [127], type I collagen and osteocalcin [128]. Lead impairs skeletal healing in a mouse model by delaying cartilage mineralisation and formation of bridging cartilage, as well as decreased expressions of collagen type II and X.
Fluoride
Fluoride is a naturally occurring mineral which can be found in ground waters as well as in fruits, vegetables, teas and other crops. In the United States, the addition of fluoride to community waters began in the 1940s after scientists noted that people living in areas with higher water fluoride levels had fewer cavities in their teeth. Water fluoridation had since been practiced by governing bodies in many parts of the world. Studies showed that the average rate of tooth decay in children was greatly reduced to by 50 % when the children drank fluoridated water [129]. Because of the positive effects of fluoride on teeth, this mineral serves as essential component in toothpastes, mouth rinses and in many other commercial products aimed to prevent tooth decay.
Once in the body, fluoride is absorbed into the blood, distributed through the blood circulation, and tends to be accumulated in areas high in calcium, such as the bones and teeth. Chronic exposure to fluoride leads to detrimental effects on these organs. Beltrán-Aguilar et al. [130] reported that the reduction in dental caries correlates with increased prevalence of dental fluorosis, characterised by defects in the forms of white streaks, spots and pitting of dental enamel. Besides, fluoride toxicity also leads to skeletal fluorosis, which is hallmarked by osteosclerosis, calcifications of ligament, as well as symptoms of osteoporosis, osteomalacia or osteopenia [131]. In addition, current evidence strongly suggests that even in the absence of skeletal fluorosis, chronic fluoride ingestion would also result in osteoarthritis [132, 133].
The exact mechanism by which fluoride causes defects in teeth and skeleton is unknown, but evidences from in vitro and in vivo studies point out the various actions of fluoride on bone cells and extracellular matrix. A study using a bone mineralising culture system which analysed the expression of bone matrix metalloproteinases (MPP) based on immunohistochemistry staining showed that fluoride alters the expression of MPP within the mineralising bone [134]. An in vivo study in rats showed excessive fluoride can inhibit the secretion of MMP-20 and disturb the balance between MMP-20 and tissue inhibitors of metalloproteinases (TIMP)-2 [135]. As a consequence, the composition of the remodelling matrix and the ensuing mineralisation process would change.
The actions of fluoride on bone cells particularly osteoblasts and osteoclasts have been well documented. In the early 1970s, it was discovered that fluoride induced new bone formation, albeit the poorly mineralised bone [136]. Later studies examining the bone markers showed that fluoride stimulates bone formation by increasing alkaline phosphatase activity [137], bone sialoprotein and BMP-2 expression [138]. Other bone formation markers including osteocalcin, Runx2, and collage type I gene expression were also elevated [139]. Osteoclastogenesis was induced in C3H inbred mouse strain when fluoride was administered in drinking water for 3 weeks, as seen by elevated levels of PTH, RANKL and TRAP5b with a concordant decrease in serum OPG [140].
In a study whereby young rabbits were given high-dose fluoride in their drinking water for 6 months, the bone turnover rate was noted to increase, with marked elevations of bone markers like bone alkaline phosphatase, serum TRAP and IGF-1. The study also showed that fluoride increased the vertebral volume by 35 % and tibial ash weight by 10 % [141].
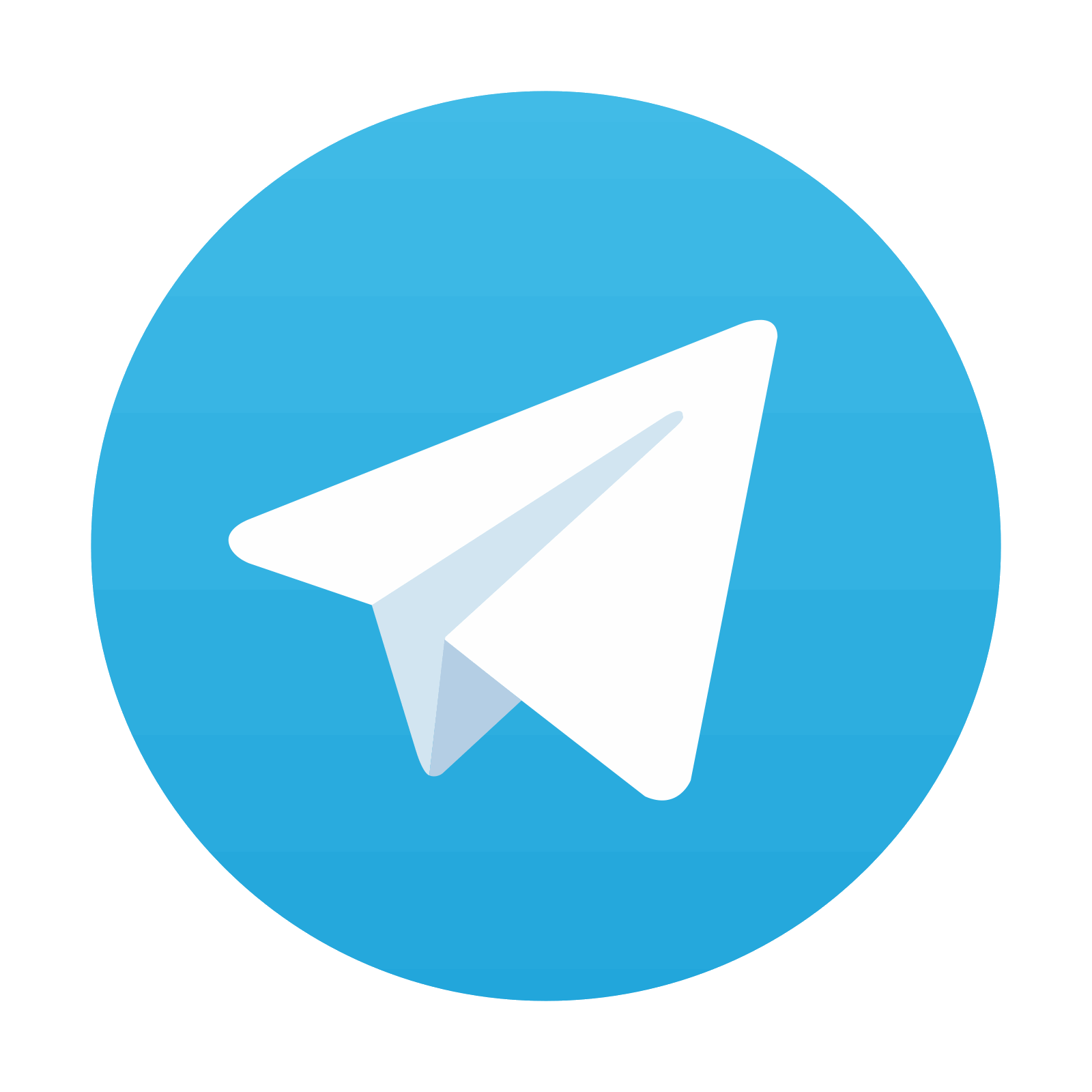
Stay updated, free articles. Join our Telegram channel
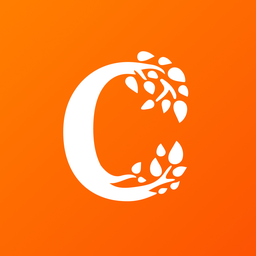
Full access? Get Clinical Tree
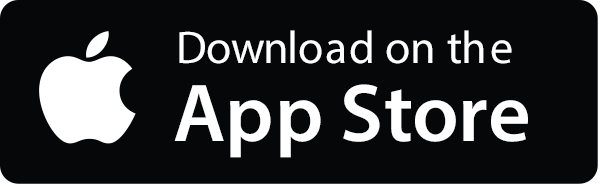
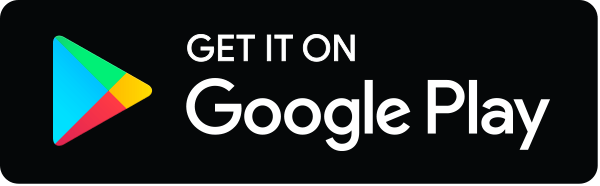