David M. Marks, PE
CONTENTS
Bioprocess Manufacturing Operations
Equipment Cleaning and Bioburden Control
Biomanufacturing Area Requirements
Implications for Performance and Compliance
Regulatory Compliance Overview
Appendix: Biopress Unit Operations
Sterilization and Part Washing
INTRODUCTION
Biotechnology is an applied science that is generally regarded as new and rapidly evolving advanced technology. Contrary to popular perception, biomanufacturing is as old as the production of fermented drink, but it has in recent decades undergone a renaissance of new applications, resulting from the development of recombinant DNA technology (Figure 12.1). Biotechnology facilities today produce a diverse array of products, from yogurt to biofuels. The focus of this chapter, however, is on the particular application of biotechnology facilities to produce products for the prevention and treatment of disease. These biopharmaceutical products are extending and improving lives and are transforming the practice of medicine at a meteoric rate.
The therapeutic products produced by biopharmaceutical facilities fall into the category of regulated substances known as biologics. The U.S. Food and Drug Administration (FDA) considers a wide range of products to be biologics, including “vaccines, blood, blood components, allergenics, somatic cells, gene therapy, tissues, and recombinant therapeutic proteins. Biologics can be composed of sugars, proteins, or nucleic acids or complex combinations of these substances or may be living entities, such as cells and tissues” [1]. Biologics may be isolated from many natural sources, including humans or animals. The focus of this chapter, however, is on biotechnology facility design for manufacturing biological products derived from microorganisms through fermentation or cell culture. An introduction to the basic principles and key concepts associated with current Good Manufacturing Practice (cGMP) biomanufacturing is covered first. Since the design of biotechnology facilities is fundamentally process driven, an overview of bioproduction systems and bioprocess manufacturing operations is provided. Additional background on bioprocess unit operations is provided in the appendix. This establishes a foundation for a discussion of the biotechnology facility programming, including area requirements, layout, and the flow of people and materials through the facility. The specific regulatory requirements that affect the design of biotechnology facilities, including cGMP and containment issues (e.g., cytotoxic and highly potent compounds and biohazardous materials), are covered. This chapter also discusses the unique challenges to the design and delivery of biotech facilities from a project management perspective. A discussion of the emerging industry trends concludes the chapter.
This chapter provides the reader with a foundational understanding of the history, technology, engineering principles, and good design practices employed to design modern biopharmaceutical plants. The strategic approach to biomanufacturing is rapidly evolving because of new technologies, such as disposable or single-use systems (SUS), continuous bioprocessing, and modular construction, all of which are discussed. The globalization of regulatory requirements and biopharmaceutical engineering standards is covered as well. The strategies being used to deliver future biomanufacturing capacity faster, better, and cheaper are presented. The next generation of biopharmaceutical manufacturing facilities will need to be more flexible and cost-effective; the objective of this chapter is to provide the reader with the concepts and tools needed to meet this challenge.
FIGURE 12.1 (a) “The Brewer,” designed and engraved in the sixteenth century (courtesy of J. Amman). (b) A technician preparing penicillin in 1943 (courtesy of Ministry of Information, Photo Division. Photographer: Stone Richard). (c) Laboratory-scale cGMP bioreactor in 2015 (Xcellerex photo courtesy of GE Healthcare Bio-Sciences Corp., Pittsburgh, PA.).
EXECUTIVE SUMMARY
OVERVIEW
Biotechnology facilities are fundamentally different from other pharmaceutical manufacturing facilities because they are required to harness the inherent complexities and variability of living things. Biologics are large and complex molecules that are difficult, if not impossible, to characterize completely [2]. In contrast to traditional pharmaceutical facilities that manufacture small-molecule drugs via chemical synthesis, biotechnology facilities produce large-molecule biologics (i.e., proteins with a molecular weight greater than 5,000 Da). For comparison, a typical monoclonal antibody (mAb) weighs 150,000 Da and contains 20,000 atoms. Most biological drugs have the ability to generate a significant immune response in the human body. Relatively small changes to the manufacturing process can have a significant effect on the efficacy or immunogenicity of the drug. In most cases, biologics are also unstable over time and require special handling and storage to protect the product from degradation. In comparison with small-molecule drugs, some manufacturing processes are more susceptible to microbial contamination. Bioburden control is required from the initial manufacturing steps through the final fill. Aseptic processing (i.e., process operations that are devoid of measurable bioburden) is required for many of these steps.
Biological therapies are the fastest-growing segment of the pharmaceutical industry. In most cases, biologics are also more profitable than small-molecule drugs due to the high cost and complexity of producing generic equivalents. Without biotechnology facilities, most of the lifesaving new drugs and revolutionary therapies introduced in the last several decades could not be manufactured.
HISTORICAL CONTEXT
Early applications of biotechnology to medicine relied on the extraction and purification of existing plant and animal sources for active ingredients. The use of microorganisms to produce drugs for medical applications in humans can be traced back to the early 1940s, when penicillin was first introduced to treat infection. Alexander Fleming’s 1928 discovery of the antibiotic properties of the mold penicillium was largely a laboratory curiosity until Pfizer (New York) opened the world’s first large-scale penicillin manufacturing facility in 1944. This facility employed deep-tank fermentation, an aseptic process for growing large quantities of microorganisms that require oxygen for survival [3]. A similar process is used to produce most commercial biopharmaceutical products today.
The scientific breakthroughs widely regarded as precursors for the modern biopharmaceutical industry are the 1953 discovery of the structure of DNA by James Watson and Francis Crick and the development of technology to transfer genetic material into bacteria by Stanley Cohen and Herbert Boyer in 1973. These discoveries led to the development of the field of recombinant DNA technology (i.e., genetic engineering), which ultimately enabled the expression of a variety of protein therapeutics through common microbial production platforms, such as Escherichia coli (Figure 12.2) fermentation. In 1978, a team of researchers from a start-up biotech company (Genentech, South San Francisco, California) and City of Hope National Medical Center, Los Angeles, developed the first viable biotechnology manufacturing process to synthesize a human protein (insulin). The resulting commercial product, Humulin, rapidly replaced animal insulin for the treatment of diabetes. In the decade that followed, several other biosynthetic manufacturing processes were developed, using recombinant DNA to introduce breakthrough therapeutics, including alpha-interferon in 1980, human growth hormone (hGH) in 1981, hepatitis B vaccine (Recombivax HB) in 1986, and tissue plasminogen activator (tPA) in 1987.
FIGURE 12.2 (a) Scanning electron micrograph of a rod-shaped E. coli bacterium; magnification, 12,800× (courtesy of CDC/Evangeline Sowers, Janice Carr). (b) A phase-contrast image of a monoclonal antibody, producing hybridoma cells grown in tissue culture. These cells are producing large amounts of monoclonal antibody, which can be readily purified from the culture media. Image taken on a Zeiss inverted microscope with 40× phase-contrast optics (photo by Gerry Shaw in the EnCor Biotechnology Lab via Wikimedia Common).
The first commercial bioprocessing used robust and fast-growing microbial production systems. However, microbial fermentation methods are not ideal for the production of some large, complex molecules. This situation led to the development of genetically engineered animal cells for the large-scale production of a new class of medicines, that is, biologics. In 1955, the first vaccine derived from mammalian cell culture was developed for polio prevention. Another significant development came with the 1975 discovery by Georges J.F. Köhler and César Milstein of hybridoma cell culture technology (see Figure 12.2) to produce monoclonal antibodies (mAbs). Cell culture is more susceptible to contamination than microbial fermentation due to a relatively long culture time in growth-promoting medium. Therefore, several advances in aseptic process technology were required before it could be implemented in large-scale cGMP manufacturing. Initially, the cell lines used were all anchorage dependent (i.e., required a substrate to attach to); thus, production was scale-limited to roller bottles or required the use of microcarrier beads in a stirred-tank bioreactor. To overcome these limitations, the ability to grow cells in suspension on a large scale was needed. By the late 1980s, suspension cell culture and aseptic process technology had developed to the extent that large-scale cell culture was a viable alternative to microbial fermentation and, in the subsequent decade, supplanted it as the predominant production system for large-scale manufacturing of new biological products.
KEY CONCEPTS AND PRINCIPLES
The design criteria for the prevention of process contamination is a central theme in this chapter. Biopharmaceutical manufacturing requires attention to the level of bioburden control that is appropriate for each step in the process. This is determined through a system of quality risk management (QRM), which ensures that the safety, identity, potency, purity, and quality of the drug substance are not compromised. The system of QRM is a regulatory requirement that involves systematic risk assessment, risk control, and risk review for every aspect of the manufacturing process, from supply train to delivery of the finished product. As it relates to the design of facilities and equipment, QRM requires particular attention to the flow of materials and personnel, and segregation of manufacturing operations, to prevent mix-ups and minimize contamination. Clean rooms, isolators, and closed process systems are employed to provide an appropriate level of protection from environmental contamination.
Contamination from bioburden is a chief concern throughout the manufacturing process. However, product contamination can also come from airborne particulates, raw materials, utility systems, product contact materials, and other products manufactured in the same facility. Therefore, the prevention of product contamination is the predominant principle driving every aspect in the design of biotech facilities. Table 12.1 presents an overview of the types of contaminants that must be considered when designing the biopharmaceutical manufacturing plant.
Contamination control is fundamentally different from containment control (as may be required for a biohazard or potent compound). Conceptually, it is the difference between protecting the product from the environment and protecting the environment from the product. Both principles require a barrier of some kind, which can lead one to confuse the requirements. Other design features, such as room pressurization gradients, may require a formal risk assessment and nuanced design discussions to identify the best method to protect both the product and personnel adequately.
TABLE 12.1
Contaminants That Must Be Considered in Biotechnology Facility Design
Source of Contamination | Potential Risk | Facility Design Impact |
---|---|---|
Materials | Viruses, bacteria, and cross-contamination | Flow of materials, controlled raw material sampling and storage areas, quality control labs, and storage of samples, product, and waste |
Manpower | Bacteria/particulate contamination and cross-contamination | Flow of personnel, gowning, and locker facilities |
Equipment and environment | Bacteria and cross-contamination | Flow of product and waste, single use, closed systems, cleaning validation, utility design, air handling and room classifications, segregation of activities, and cleanable surfaces |
FIGURE 12.3 Open and closed processing.
One determining factor in the assessment of risk in biomanufacturing is the nature of process isolation from the environment. An open process has no physical barrier between the product and the local environment and relies heavily on clean room conditions to mitigate the risk of contamination. Critical open process operations are often performed in biosafety cabinets (BSCs) or restricted access barrier systems (RABs). Open processing is inherently less reliable than processing in a closed system, which relies on equipment to isolate the product from the environment (Figure 12.3). A closed system is defined as a “process system that is designed and operated such that the product is never exposed to the surrounding environment” [4]. When biomanufacturing is executed entirely in closed systems with material transfers performed in a completely closed fashion, a less stringent area classification may be employed.
Many bioprocess systems are routinely opened to prepare the equipment for processing, and then rendered closed through a validated cleaning and sanitization or sterilization step before process use. Such process systems are frequently referred to as functionally closed [5]. In some briefly exposed operations, such as buffer and media preparation, material is initially processed in an open bioburden-controlled environment and then rendered closed for subsequent process operations (Figure 12.4).
DEFINITIONS
An understanding of the contamination control terminology used to describe bioprocessing operations is critical to identify the design requirements for a biotechnology facility. Conversely, an incomplete understanding of these terms can result in facility features that are either inadequate or unnecessary. Even within the biotech industry, the terminology can be confusing. For example, some operations are described as sterile or sterilized; these terms do not, in this context, refer to the complete absence of life. In practice, the process of rendering something sterile, or sterilization, refers to a condition where the probability of remaining bioburden (i.e., number of viable contaminating microorganisms present) is statistically insignificant. Most bioprocessing unit operations are bioburden controlled but not truly sterile. Often, the sterilization of equipment is used to assist in bioburden control.
FIGURE 12.4 Briefly exposed and functionally closed processing.
The most accurate term for describing bioburden-free operations in biotechnology facilities is aseptic. Aseptic operations are considered devoid of detectable bioburden. Processing in bioreactors is occasionally described as aseptic but is more accurately described as axenic. The term axenic refers to a culture that contains a single strain of living organism (as intended in a bioreactor) but is entirely free of all other contaminating organisms.
The manufacture of drug substances from living organisms is often referred to as bioprocessing. Bioprocessing requires clean manufacturing under conditions of low bioburden. The term hygienic, as used in the biopharmaceutical industry, refers specifically to the maintenance of cleanliness so that the product does not adversely affect human health.
BIOTECHNOLOGY FACILITY DESIGN
A proper approach for biotechnology facility design starts with a thorough understanding of the manufacturing process (i.e., the process flow, the timing of operations, and the equipment and utilities that are required). From this foundation, designers can proceed with architectural programming, including adjacencies and transitioning to cGMP areas, to lay out the facility.
PRODUCTION SYSTEMS
The user requirements for biotechnology facilities are heavily influenced by the type of product (e.g., protein therapeutics, mAbs, vaccines, gene therapy, or stem cells), the propagation system (i.e., host microorganism used to produce product), and the final dosage form. Most biopharmaceutical products are delivered as a parenteral (i.e., injectable) dosage form, whereby the drug is injected directly into the bloodstream; thus, the level of contamination prevention and bioburden control required is much more stringent than that required for oral or topical dosage forms. Bioburden is a particular issue with injectable drugs for the following three key reasons:
Endotoxins created by Gram-negative microbial contaminates can elicit an immune response with unwanted side effects (e.g., inflammation, fever, internal bleeding, and septic shock).
Exotoxins from environmental bacteria, which are not routinely monitored, can cause cell culture death and are highly toxic to humans in small quantities.
Bacterial action on the product may cause unwanted variants by clipping protein chains or changing the glycosylation pattern (neither of which may be readily detectable by analytical testing).
Product contamination can come from airborne particulates, raw materials, utility systems, product contact materials, and other products manufactured in the same facility. Therefore, the prevention of product contamination is the predominant principle driving every aspect in the design process of biotech facilities.
Biopharmaceutical products are diverse and are produced by a variety of methods. Therapeutic proteins and mAbs are typically produced by microbial or cell culture propagation systems. The first commercial biopharmaceutical products used microbial fermentation (e.g., bacteria, yeast, and fungi) for protein production. These simple and fast-growing production systems produce a high yield, but usually have the disadvantage of intracellular product expression. Since in this case the product is produced inside the cells, the cells must be lysed (i.e., ruptured) as part of the manufacturing process to release the protein being produced. Unfortunately, this process also releases cell debris from the host cell, which requires relatively extensive purification to reduce impurities to safe levels in the bulk product. A cell culture production system, on the other hand, has the advantage of extracellular expression. During cell culture, product expression is by secretion of protein through the cell wall. Instead of lysing the cells to harvest product, the product is drawn from the media surrounding the cells. This significantly simplifies the process purification requirements. In addition, cell culture is capable of producing the biologically active pharmaceutical ingredient (API) in a form that is more usable by the human body, which also simplifies downstream processing and improves product potency. Cell culture production systems are usually based on mammalian cell lines, such as Chinese hamster ovary (CHO) and hybridoma cells, although some cultures use insect or plant cells. Both microbial and cell culture protein production systems are in common use today, although microbial propagation systems are best employed for production of simple proteins that do not require significant posttranslational modifications to activate the product. This discussion is relevant to the topic of biotechnology facility design because the choice of propagation system has a significant impact on the manufacturing process, which, in turn, affects the equipment requirements, process utilities, area classification, flows, and space planning for the facility.
Vaccine manufacturing, originally produced from live animals or fertilized eggs (Figure 12.5), involves the production of an antigen that triggers an immune response in the patient. Today these products are also manufactured using microbial and cell culture production platforms. The method of production has a huge impact on facility design, particularly if the manufacturing process uses live viruses, which must be processed in a segregated area.
BIOPROCESS MANUFACTURING OPERATIONS
In comparison with other chemical process industries, unit operations for biologics manufacturing are typically executed on a relatively small scale. Following the commercialization of blockbuster drugs in the first couple of decades after the introduction of recombinant DNA technology, biopharmaceutical manufacturers became increasingly reliant on product portfolios that include drugs for small patient populations (requiring small volumes of product per batch). In addition, optimized biomanufacturing has yielded improvements in the process titer over the past decade, further reducing the manufacturing scale required for many high-value biologics.
FIGURE 12.5 Flu vaccine production: eggs being inoculated with the seed virus (Val de Reuil, France, March 2009). (Courtesy of Vincent Moncorgé; copyright Sanofi Pasteur, Lyon, France.)
Batch processing has historically dominated biotechnology manufacturing because of available technologies, risk aversion, and perceived regulatory difficulties associated with continuous manufacturing. The industry typically relied on existing batch technology to provide a safe and reliable process. However, emerging technology has opened up many options to make continuous processing more feasible in drug manufacturing. Bioprocess development for future facilities is expected to make greater use of continuous processing to enable more efficient manufacturing on a small scale.
As bioprocessing is a wet operation requiring frequent cleaning, cGMP bioprocess equipment is typically designed and constructed in a manner that facilitates external cleaning and sanitization. Likewise, the manufacturing areas that are designed to accommodate surface cleaning and the occasional spill typically have a floor drain, berm, or other architectural feature designed to collect fluids. Most bioprocess unit operations are aqueous processes, although small quantities of solvents may be used for downstream processing (e.g., organic solvents in operating buffers for some chromatography steps), which may require explosion-proof areas designed to handle flammables and combustibles safely.
Biotechnology manufacturing operations are typically categorized as upstream or downstream processes (Figure 12.6). Upstream bioprocessing refers to all of the manufacturing processes required to produce the biological APIs, including inoculum preparation, bioconversion (via fermentation or cell culture), and harvest steps (via centrifugation or filtration). Downstream bioprocessing refers to all of the processing required for the API to meet purity and quality requirements, including product recovery, purification, and polishing steps (via chromatography and filtration). After downstream processing, the purified drug substance is formulated (sometimes also conjugated) and filled into a bulk container for storage in a stable form. Upstream, downstream, and bulk filling processes are all supported by ancillary manufacturing activities, including weigh and dispense, media preparation, buffer preparation, sterilization, and part washing operations. Refer to the Appendix for a more detailed discussion of bioprocess unit operations and their relevance to good design practice for biotechnology facilities.
FIGURE 12.6 Typical biotechnology manufacturing operations.
FIGURE 12.7 Typical central CIP system. (Courtesy of Sani-Matic, Inc., Madison, WI.)
EQUIPMENT CLEANING AND BIOBURDEN CONTROL
Traditional biomanufacturing in stainless steel equipment requires facility infrastructure for cleaning and sterilization. Centralized clean-in-place (CIP) systems (Figure 12.7) are designed to prepare and distribute cleaning solutions throughout the plant to facilitate cleaning of equipment in situ. These systems are typically designed to recirculate cleaning solutions through the product contact areas of the process equipment, although single-pass systems may be required for cleaning areas with potential biohazard exposure. The design of distribution loops for CIP is critical because it requires piping that is correctly sloped and free of dead legs (i.e., pockets with stagnant areas). The CIP rinsing operations are typically the largest user of compendial water in a facility, so system drainability and minimizing holdup are important for economical and sustainable water use. This places some constraints on facility design, whereby it is often desirable to locate CIP systems in mechanical space near the equipment to be cleaned. In multilevel facilities, it may be advantageous to locate CIP areas in the space above or below the process to facilitate the drainability of distribution piping. For some small bioprocess systems, cleaning may be accomplished with temporary connections to portable CIP systems (Figure 12.8) or through disassembly and cleaning-out-of-place (COP) (Figure 12.9).
FIGURE 12.8 Portable CIP station. (Courtesy of ABEC, Bethlehem, PA.)
FIGURE 12.9 Typical COP station. (Courtesy of Electrol Specialties Company (ESC), South Beloit, IL.)
The number of CIP systems required for biomanufacturing is determined by production timing and process segregation requirements. As CIP systems can be a source of cross-contamination, separate systems are typically used for buffer or media preparation and upstream and downstream operations. Further process segregation may be required as determined by QRM assessment.
FIGURE 12.10 Pure steam generator without steam distribution piping. (Courtesy of STERIS FINN-AQUA, Tuusula, Finland.)
For equipment sterilization or sanitization, centralized clean steam systems (Figure 12.10) are typically connected directly to process equipment for automated steam-in-place (SIP) operations. In addition, SIP and CIP stations may be required to make temporary connections to mobile tanks or process systems for equipment sanitization, sterilization, or cleaning.
SINGLE-USE TECHNOLOGY
Single-use technology may be used for biomanufacturing in which materials in contact with the process are discarded after processing. This technology uses gamma-irradiated bags, connectors (Figure 12.11), and tubing (Figure 12.12) to enable aseptic closed processing without CIP or SIP requirements (Figure 12.13). The capital equipment investment for SUS is less than that for comparable stainless steel systems, but operating costs for consumable supplies and waste disposal are higher.
FIGURE 12.11 Typical single-use connectors: upper left, MPX quick connect; upper right, connected MPX connectors; lower left, aseptic connector family; lower middle, unconnected genderless aseptic connector pair; lower right, steam-through connectors. (Courtesy of Colder Products Company, St. Paul, MN.)
FIGURE 12.12 Tubing and attachments are critical aspects of single-use technologies: upper left, MPX and MPC quick connectors with tubing; upper right, aseptic quick and steam-through connectors connected to a tank assembly; and lower left, unconnected genderless septic connector pair with tubing. (Courtesy of Colder Products Company, St. Paul, MN.)
If single-use technology is deployed extensively in a facility, a significant reduction or elimination of CIP or SIP infrastructure requirements may be possible. Relative to traditional facilities that use stainless steel tanks and process piping, SUS technology also provides the advantages of faster turnaround times for process systems (increasing throughput), a reduction in compendial water and waste neutralization requirements, and greater process flexibility for multiproduct manufacturing. In addition, single-use technology may be used to simplify and facilitate closure of a process, thereby reducing facility segregation requirements and enabling manufacturing in controlled nonclassified (CNC) space or areas of lower classification. Reported benefits of this approach include a reduction in manufacturing area requirements by 15%–30%, a reduction in clean utility requirements by 80%–90%, and reductions in steam and chilled water requirements by up to 60% [6].
FIGURE 12.13 Aseptic closed processing with single-use or disposable technology. (Courtesy of FUJIFILM Diosynth Biotechnologies, Morrisville, NC.)
Single-use solutions are commercially available for almost every step in biomanufacturing (excluding only unit operations that require pressure or temperature extremes or handling of organic solvents). Manufacturing with SUS requires a commitment to robust supply train management to ensure the consistent quality of consumables (Figure 12.14). The handling for installation and removal of bioprocess bags is also critical to maintaining the integrity of SUS. Facilities that make extensive use of SUS will require additional space for controlled-environment storage and staging of consumables, as well as collection and disposal of disposables after use. Areas that use SUS in contact with biohazardous materials may require a decontamination autoclave to process disposable waste before it can be removed from classified space.
The application of SUS is limited by process scale. Manually installing and removing large bags, particularly when wet, is generally very difficult above a 2,000 L scale. Likewise, it is difficult to safely move materials in bags greater than 500 L due to limitations associated with the weight of materials being manually manipulated. Larger mobile containers are available, but they may require the use of motorized pallet jacks for safe handling. There are also limitations to the size of commercially available process transfer tubing that can facilitate aseptic connections.
Process suites designed for flexible use of mobile SUS may provide ceiling-mounted utility panels to facilitate easy connections to electrical power and process utilities at multiple stations within the processing area. The facility layout should take into account the most likely combinations of equipment arrangements and ensure that there is adequate space for the staging of material transfer bags. Equipment arrangements and adjacencies should also minimize the length of transfer tubing required between unit operations.
PRODUCT TRANSFER
The method of material transfer in a bioprocess facility has a large impact on its design. In production-scale facilities with hard-piped product transfer, a large network of highly automated process piping, requiring SIP and CIP, is needed. This may require a complex system of transfer panels and automated valve clusters for routing and isolation of process fluids. The impact on facility architecture and mechanical space requirements can be significant. Therefore, front-end process design is a critical component of space programming.
FIGURE 12.14 Typical SUS supply chain based on a single-use bioreactor example. Note that the outsourced components usually are from multiple vendors and manufacturers.
Facilities that rely heavily on single-use technology may eliminate much of the process piping infrastructure in a facility, but instead bring a relatively larger impact on loading docks, storage, and material transfer areas of the facility. Aseptic connections with single-use tubing are typically accomplished via a sterile tube welder or aseptic disposable connector. Material transfer between processing areas is usually executed by manual manipulation of mobile bag containers through airlocks and hallways, so the traffic in these areas may require additional consideration.
Where large volumes of process fluids are transferred between unit operations via disposable tubing, consideration should be given to the routing and support of flexible tube lines. The flexible tubing interconnecting SUS can create contamination and personnel safety issues if allowed to rest unsupported along the floor. The provision of cleanable racks or hangers to support flexible tubing is a good design practice. These may be mounted to the walls or ceiling to facilitate tubing support overhead. If disposable tubing is used to pass fluids between classified areas (e.g., bioreactor media charging), a sealed “mouse hole” or clean room pass-through system will be needed to feed the tube through the wall of an adjacent classified area.
PROCESS AUTOMATION
At the unit operation level, most process automation applications for batch processes are not complex. Automated system reliability and the integrity of data captured for the batch record are of paramount importance for cGMP manufacturing. This requires an automation development process that is highly structured, well documented, and validatable. Beyond the rock-solid robustness that is required for all cGMP pharmaceutical manufacturing operations, process analytical technology (PAT) is recommended by regulatory authorities to provide “a system for designing, analyzing, and controlling manufacturing through timely measurements (i.e., during processing) of critical quality and performance attributes of raw and in-process materials and processes, with the goal of ensuring final product quality” [7].
Bioreactor control systems present one of the more difficult bioprocess automation challenges because batch automation is required to accommodate a large load variance throughout the culture life cycle, from lag phase through exponential growth. Control parameters may be adjusted, according to bioreactor growth phase, by using time profiles or adaptive control to adjust for the anticipated growth curve and inherent variability of living organisms.
The next level up from basic process automation of unit operations is supervisory control and data acquisition (SCADA). The SCADA system provides a method to access equipment status, ongoing operations, and process variables for the entire manufacturing process. Human–machine interface (HMI) stations may be provided within clean manufacturing space to allow operators SCADA access to view and control local operations, as well as critical utilities and processes in other manufacturing areas. Since access to clean room areas is restricted and requires special gowning, control rooms are typically provided for personnel to view the process, respond to alarms, and access the SCADA data historian.
In traditional biomanufacturing facilities, the most challenging automation requirements usually come from the need for precision control of the sequential steps required for CIP and SIP of process systems. These automated operations require the concurrent, coordinated control of multiple unit operations and must mediate the usage of shared CIP systems and transfer lines. CIP and SIP automation can provide a safe and highly reliable cleaning and bioburden reduction capability with very little manual intervention.
Single-use systems require far less plant automation because the cleaning and sterilization of product contact services are essentially outsourced to the SUS vendor. However, the performance and reliability of these systems are far more dependent on manual manipulations (see Figures 12.11 and 12.12). In such facilities, the manipulation of SUS is a key component of the operator training program. Further risk reduction can be provided through automated materials tracking and tracing, including the many disposable components that are required for the SUS manufacturing process. Manufacturing execution systems (MESs) are the next level above SCADA and typically provide this function.
Process automation is a key enabler of continuous bioprocessing in regulated biotechnology facilities. Continuous processing in a cGMP manufacturing environment requires continuous monitoring and PAT, which enable parametric release. Parametric release (real-time release) is a quality assurance release program where demonstrated control of the process enables a firm to use defined critical process controls, in lieu of final quality control testing, to fulfill the intent of regulatory release requirements, such as 21 CFR §211.165 and §211.167. Essentially, PAT provides continuous assurance that a process is working correctly and that the product is of the right quality throughout the process.
PROCESS UTILITIES
The process utilities used in a biotechnology facility include general plant utilities, such as plant steam, chilled water, and compressed air, as well as clean utilities, such as compendial water, clean steam, and clean gas systems. The QRM method requires a criticality assessment of process utilities as part of facility design to identify the impact of utility systems on product quality. The reliability of process utility systems in cGMP biotechnology facilities can be every bit as critical as that of the process equipment for unit operations. Fermentation and cell culture operations can require sustained utility services for days, weeks, or months at a time. Therefore, system reliability, equipment redundancy, and ease of maintenance are key considerations during system design to mitigate the risk of failure and its impact on the product being produced. Biotechnology facilities are typically designed with backup electrical and mechanical systems for this reason (e.g., uninterruptible power supply [UPS] power, backup generators, redundant pumps, redundant boilers, and air compressors).
As cGMP biotechnology facilities typically produce parenteral products, the clean utility systems that have a direct path to product contact surfaces or that have the potential to come into direct contact with the product must be designed to operate free of contaminates that could be carried into the product stream. These systems are typically designed for sampling at use points to verify the consistent quality of clean utilities supporting the process. Unless the process is small enough to be supported by outsourced compendial water supplies, a utility system is typically required to generate, store, and distribute water for injection (WFI) for process formulation and for equipment and parts’ final rinse during cleaning.
BIOMANUFACTURING AREA REQUIREMENTS
Biotechnology facilities have distinctive areas for manufacturing and manufacturing support that can be characterized in terms of function, adjacencies, and typical user requirements (Figure 12.15). Depending on the spatial segregation and environmental requirements of the process, each of these areas may represent a separate room or, under certain conditions, several operational areas within a room. Typical operational areas for biomanufacturing are described in Table 12.2.
In addition to the core manufacturing areas typical to biotechnology facilities, several manufacturing support areas are necessary for cGMP operations. These are summarized in Table 12.3.
FACILITY SPACE PROGRAMMING
Programming is a systematic process for decision making about organization and project values, goals, and requirements [8]. This is the process whereby required facility spaces are identified, and the size and relationships between these spaces are established.
FIGURE 12.15 Overview of typical area adjacencies for biotechnology manufacturing.
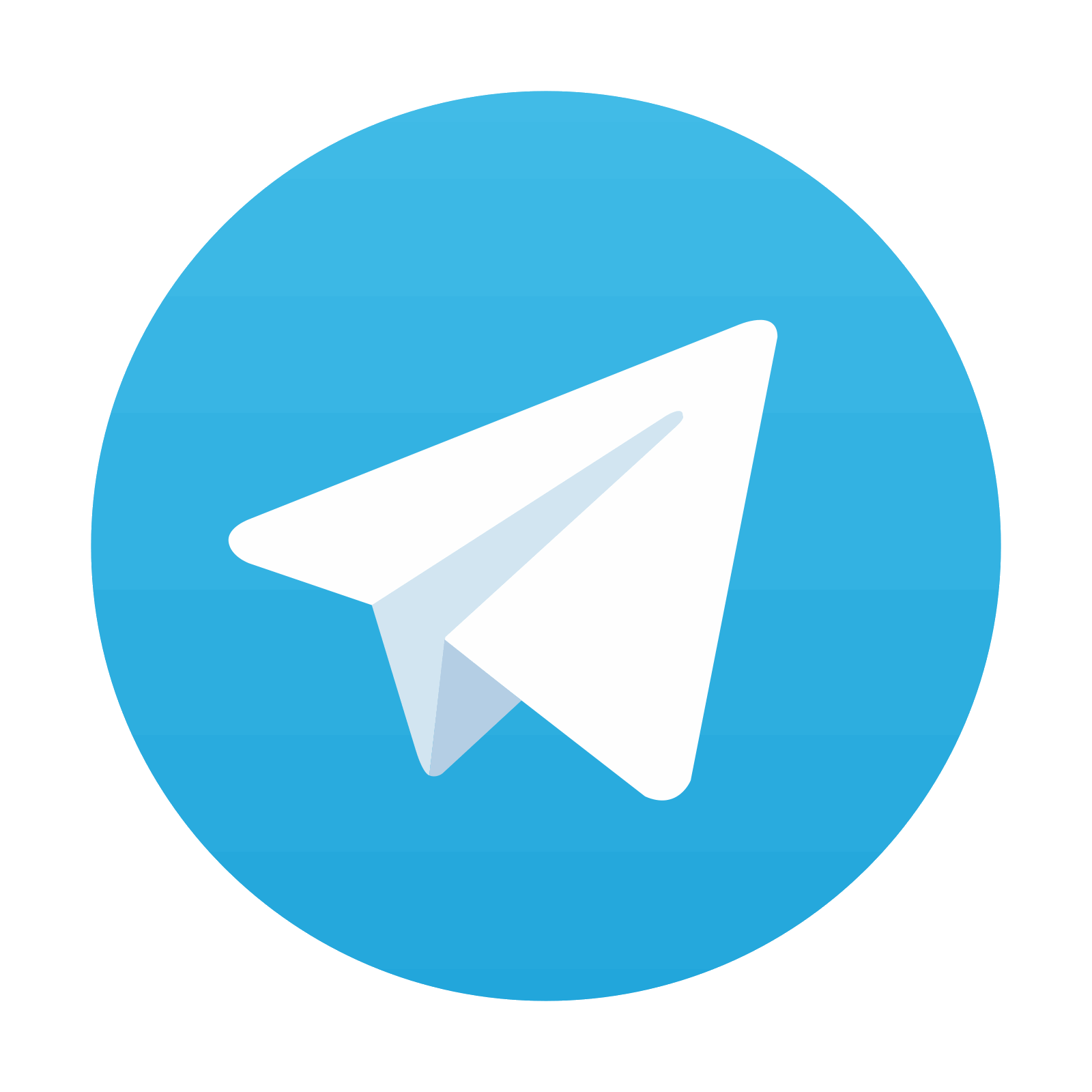
Stay updated, free articles. Join our Telegram channel
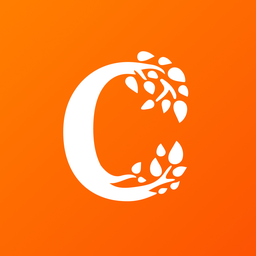
Full access? Get Clinical Tree
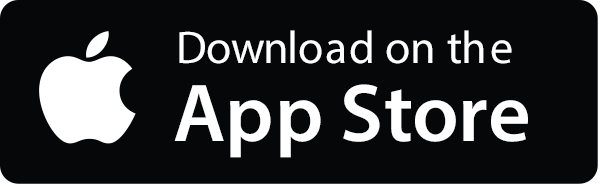
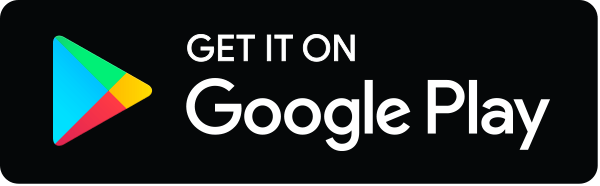