Method category
Method
Size
Pros and cons
Methods references
Size-exclusion chromatography
HPSEC
<0.1 μm
Pros: fast, high throughput
Cons: limited size range, limited choice of buffers, possible matrix interactions
Field-flow fractionation
AF4
<1 μm (normal mode) and 1–50 µm (steric mode)
Pros: high throughput, broad dynamic range, works with wide range of buffers
Cons: requires highly trained analysts. Membrane interactions are possible. Separation mode crossover at ~1 μm
Sedimentation
AUC
<0.1 μm
Pros: works with wide range of buffers and conditions, no matrix interactions
Cons: low throughput, requires highly trained analysts
Light scattering
Static light scattering
<1 μm
Pros: absolute measurement
Cons: requires highly trained analysts, does not resolve different sizes
Dynamic light scattering
<1 μm
Pros: fast, easy, can be high throughput
Cons: sensitive to dust, does not resolve different sizes in heterogeneous samples
Turbidity
NA
Pros: compendial test, fast, easy
Cons: no quantitative information on size, counts
Tatford et al. (2004)
Nanoparticle tracking analysis
0.02–1 μm
Pros: unbiased size measurements in heterogeneous samples
Cons: limited dynamic range (~107–109 particles/mL)
Filipe et al. (2010)
Light obscuration
Light obscuration
1–150 μm
Pros: compendial test. Counts one particle at a time, does not rely on distribution models
Cons: sizing of protein particles is not reliable. Does not work well with high protein concentrations, turbid and viscous samples
Electrical sensing zone
Electrical sensing zone
0.4–1,200 μm
Pros: wide dynamic range, counts single particle at a time
Cons: limited concentration range, requires electrolyte solution and multiple measurements with different apertures to cover the entire range
Narhi et al. (2009)
Microscopy
EM
<100 μm
Pros: high resolution
Cons: sample preparation artifacts possible
Roux (1999)
AFM
<1 μm
Pros: high resolution
Cons: sample preparation and imaging artifacts possible
Light microscopy
>0.5 μm
Pros: fast, easy
Cons: low precision, difficult to detect translucent particles
Barber et al. (1989)
Flow microscopy
Flow microscopy
>0.5 μm
Pros: fast, provides information on counts, size, and morphology
Cons: difficult to detect translucent particles
Mass spectrometry
Electrospray or MALDI MS
>0.1 μm
Pros: detailed structural information, high sensitivity and resolution
Cons: requires highly trained analysts. Potential dissociation of non-covalently linked aggregates Limitation of m/z range. Qualitative or semiquantitative method
New technology
Gas-phase electrophoretic mobility
0.003–0.25 μm
Pros: fast, direct measurement, high resolution
Cons: limited concentration range, electrospray buffer different from formulation buffer
Pease et al. (2008)
Resonant mass measurement
>0.1 μm
Pros: measures single particle at a time, direct mass measurement, can distinguish different types based on density
Cons: low sensitivity to particles with density close to that of surrounding solution
Burg et al. (2007)
4.2 Analytical Methods for Size Distribution Analysis
4.2.1 Size-Exclusion Chromatography
High-performance size-exclusion chromatography (HPSEC) is one of the most frequently used analytical methods for detection and quantitation of soluble protein aggregates and fragments. High-throughput capability and a good resolution between monomers and soluble aggregates are important advantages of HPSEC.
HPSEC separates molecules based on partitioning between porous stationary phase and aqueous mobile phase. The protein molecules are eluted according to their hydrodynamic size rather than actual molecular weight. Monomeric conformational variants of monomer may have different hydrodynamic sizes and, therefore, elute as separate peaks. For example, partially denatured monomers with an elongated shape have been shown to elute before the monomer peak (Philo 2006). Protein glycosylation with large oligosaccharides often affects hydrodynamic size and can have a noticeable effect on the peak position in the HPSEC profile (Wen et al. 1996).
Reversible self-association can be detected by HPSEC as either broadening of monomer peaks or through the presence of shoulder peaks. Although HPSEC can be useful for analysis of protein self-association (Moore et al. 1999), the results should always be interpreted with caution. In some cases nonspecific interactions with the column matrix can cause distorted peak shape (Wang et al. 1996).
Other limitations of HPSEC include the potential loss of some aggregate species due to selective absorption on the stationary phase (Liu et al. 2006; Gabrielson et al. 2007a) or retention by the column of very large aggregate species exceeding the upper HPSEC limit of about 0.1 µm. In addition, higher ionic strength, choice of salts, or different pH of HPSEC mobile phase may change protein conformation, induce new aggregates, or cause dissociation of weakly associated aggregates and thus bias the results (Washabaugh and Collins 1986; Philo 2006).
4.2.2 Field-Flow Fractionation
Asymmetric flow field-flow fractionation (AF4) is an important orthogonal separation-based matrix-free technique with a wide dynamic range, capable of analysis of fragments, monomers, and aggregates up to 50 μm in size (Gabrielson et al. 2007a; Litzen et al. 1993; Liu et al. 2006; Cao et al. 2009a). AF4 separation is carried out in a flat trapezoidal channel equipped with a semipermeable membrane. Analytes are separated based on their hydrodynamic size by a cross flow through the semipermeable membrane, perpendicular to the elution flow. The pore size of the semipermeable membrane is selected to retain the analytes, but it allows a free flow of the mobile phase. In the typical AF4 separation, the elution flow is the fastest in the middle of the channel and slower near the top and the bottom. Cross flow pushes larger species closer to the semipermeable membrane at the bottom of the channel; therefore, larger species elute later, after smaller species. Recent publications demonstrated that AF4 can be successfully applied for analysis of large protein aggregates, and collected data are consistent with other aggregate analysis methods such as HPSEC and flow microscopy (Cao et al. 2009b).
One of the important advantages of AF4 is a wider selection of mobile phases compared to HPSEC, because the former is compatible with a broader range of pH and salt concentrations. Other advantages include a wide dynamic range for separation of different species and the absence of a stationary phase.
Limitations of AF4 include potential nonspecific interactions of certain analytes with the semipermeable membrane, lack of information on particle counts, and a crossover of the separation mode in the 1 μm range described below. Nonspecific interactions of analytes with the semipermeable membranes may lead to distorted elution profiles. The possibility of such interactions should be carefully assessed for each sample type. If needed, mobile phase should be optimized to minimize the nonspecific interactions. Separation mode crossover may pose a significant challenge for AF4 analysis of highly heterogeneous samples. For species larger than 1 μm, the steric elution mode becomes dominant. It results in a faster elution of larger analytes, which is the opposite of the normal elution order in the typical AF4 profile (Yohannes et al. 2011). Therefore, the analysis of samples containing both sub- and above-micron species can be compromised, which can limit AF4 practical applicability to submicron range.
4.2.3 Analytical Ultracentrifugation
Analytical ultracentrifugation (AUC) is widely used for measurements of aggregate and fragment levels, determination of aggregate stoichiometry, and analysis of reversible self-association (Liu et al. 2006; Gabrielson et al. 2007b; Rivas et al. 1999). AUC can also provide information on distribution of protein conformations, if they are related to different molecular shapes. Two major AUC methods are sedimentation velocity and sedimentation equilibrium. In a typical sedimentation velocity experiment, AUC cell channels loaded with a sample and a reference buffer are scanned by either absorbance or interference optics as analytes sediment to the bottom of the cells at relatively high speed, about 45,000 rpm. Collected scans contain information on the shape of the sedimentation boundary as a function of time. Sedimentation boundaries depend on both the size and shape of the sedimenting molecules. Molecular weight distribution can be derived from the analysis of multiple sedimentation boundaries if certain assumptions about the shape of the molecule can be made.
Sedimentation equilibrium experiments involve spinning at lower speeds until sedimentation-diffusion equilibrium is established. Usually, several different speeds and sample concentrations are evaluated in one sedimentation equilibrium experiment. Molecular weight distribution information is derived from the analysis of scans by fitting to theoretical distribution models (e.g., monomers, monomers and non-dissociable aggregates, reversible dimers, and trimers).
Similarly to AF4, important advantages of AUC are the minimization of matrix interactions, which may result in loss of some aggregate species, and compatibility with many buffers in a broad pH range. In addition, AUC allows sample analysis at predetermined concentrations, and it is the method of choice for studying self-association behavior. Limitations of AUC include the following: a relatively high (about 1 %) limit of quantitation for small aggregates and fragments, a relatively narrow (up to approximately 0.1 μm) dynamic range, low throughput, and the requirement for highly trained analysts (Narhi et al. 2009).
4.2.4 Light Scattering
Multiple static and dynamic light scattering techniques are widely used for protein size heterogeneity analysis, including sizing and quantitation of aggregates (Follmer et al. 2004; Philo 2006, 2009), distribution of glycosylation (Wen et al. 1996), and reversible self-association (Wu et al. 1997; Attri and Minton 2005). In methods based on static or classical light scattering, the molecular weight of molecules in solution is derived from the light scattering theory equations. These equations link scattered light intensity and concentration to the molecular weight. Multiangle light scattering (MALS) applications are most commonly used for proteins in solution (Oliva et al. 2004). In a typical MALS measurement, light scattering intensity measured at three or more angles is determined and used to calculate the molecular weight. For molecules larger than 10 nm, the radius of gyration can be also calculated based on the dependance of the light scattering intensity on the scattering angle.
Dynamic light scattering (DLS) relies on the analysis of an autocorrelation function derived from measurements of scattered light intensity fluctuations due to Brownian motion of molecules in solution (Jossang et al. 1988). The translational diffusion coefficient D τ of protein molecules in the samples is derived from the autocorrelation function. The hydrodynamic radius R h is calculated using the Stokes–Einstein equation linking D τ, temperature, and viscosity of the solution.
Both static and DLS methods can be used to determine the presence of protein aggregates in solution and to measure aggregates up to 1 μm in size, including high-throughput screening applications. A composition gradient technique based on static light scattering measurements of increasing protein concentrations can be applied for analysis of self-associating systems (Attri and Minton 2005).
The light scattering methods work particularly well for sizing of monodisperse samples. However, they have significant limitations in the analysis and quantitation of size distributions in polydisperse samples. Results can be skewed to larger sizes, because scattering intensity depends on the sixth power of the size, and thus, larger species scatter significantly more light. If separation of different species (e.g., by HPSEC or AF4) is carried out prior to analysis, it significantly improves the sizing capabilities of the light scattering methods. MALS or DLS coupled with HPSEC or AF4 as on-line detectors are powerful methods for the characterization of aggregates. In particular, MALS and DLS detectors in tandem can provide information on the molecular weight, size, and shape of aggregates (Rambaldi et al. 2011). Proteins with high levels of glycosylation can be analyzed with a triple-detector HPSEC method with consecutively attached MALS, UV, and refractive index detectors. This method allows on-line determination of proportions and distributions of oligosaccharide across different fractions (Wen et al. 1996).
Solution turbidity measurement is a low-resolution light scattering-based technique that can provide a rough assessment of size heterogeneity levels. Solution turbidity can be determined quantitatively by intensity loss of the transmitted beam or by the intensity of the light scattered at a given angle. The presence of protein aggregates or particles often results in an increase in turbidity. Therefore, the degree of turbidity in a protein solution may be used to qualitatively assess the extent of aggregation (Tatford et al. 2004).
Nanoparticle tracking analysis (NTA) is a new light scattering-based technique that is rapidly gaining use for the analysis of submicron particles (Filipe et al. 2010). Particle size measurements are based on tracking the distance traveled by individual particles over a given amount of time and do not rely on the scattering intensity. Due to this, the NTA technique can provide relatively unbiased measurements of particle sizes even in samples with heterogeneous populations. A rather narrow dynamic range of particle counts suitable for the measurements (~107–109 particle/mL) is a limitation to its applicability.
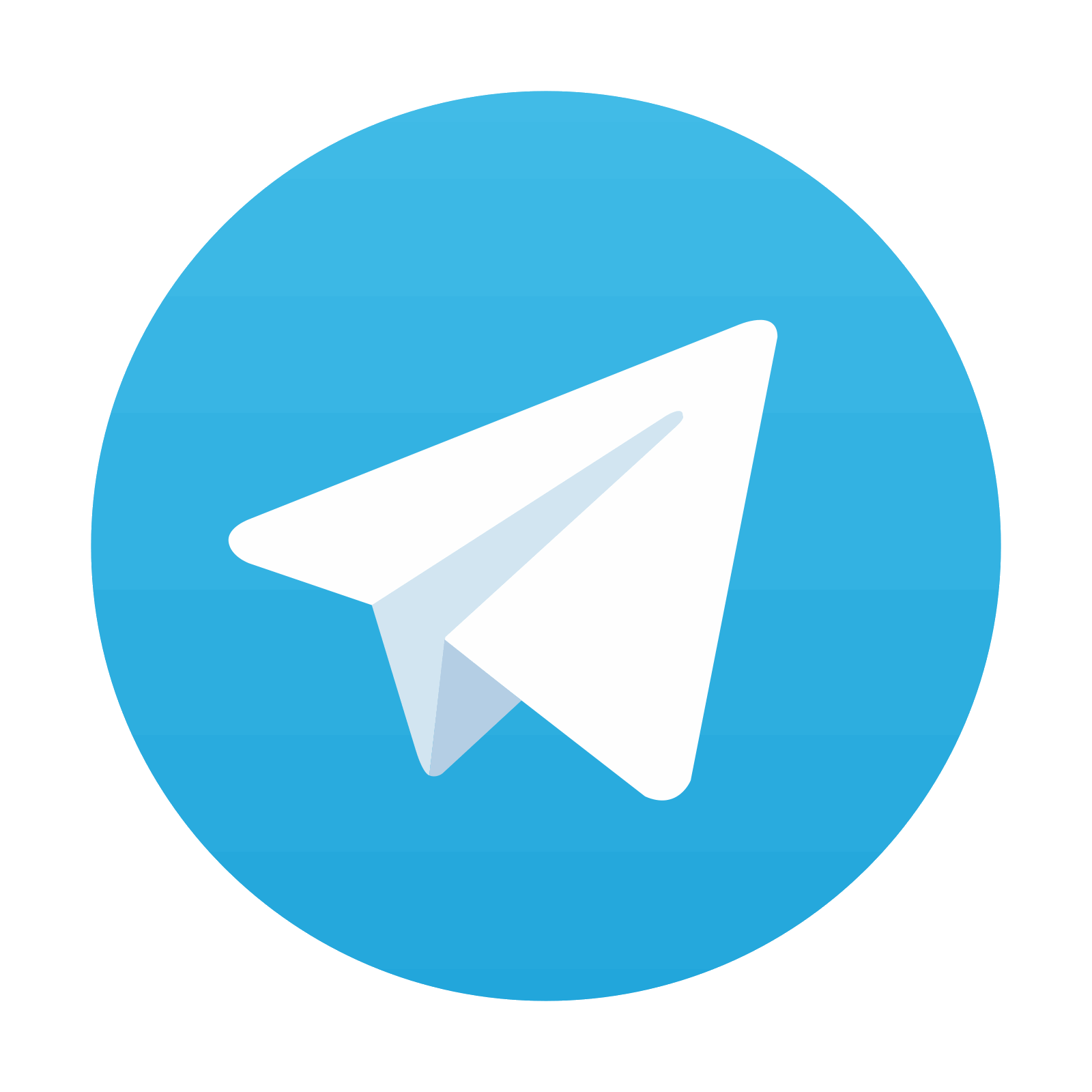
Stay updated, free articles. Join our Telegram channel
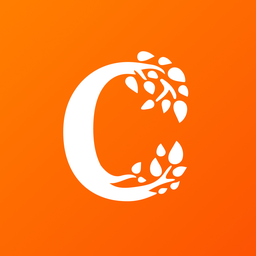
Full access? Get Clinical Tree
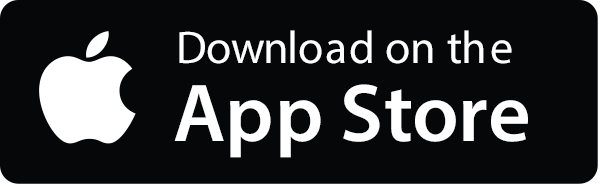
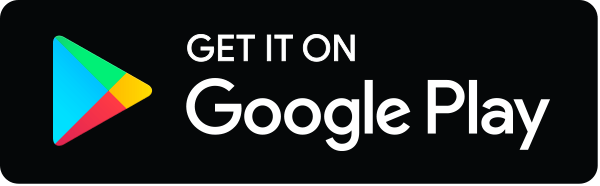