Fig. 1.1
Anatomic structure of knee joint
The hip joint is a classic example of the ball articular joint with three degrees of freedom. It is a compact union of the femoral head almost spherically shaped ball and the pelvic acetabulum shaped hemispherically that form a multiaxial system with an extensive scope of motions. Therefore, the joint in the average physiological position is capable to contract and extend in the sagittal plane within 140°, to retract and extend the extremity in the frontal plane within e 50°, to rotate it around the hip longitudinal axis through 50° in the horizontal plane. The motions are limited by the tension in the capsule, ligaments and muscles and can vary considerably in response to the joint spatial position, e.g., when the hip is bent, moved away, etc.
The knee joint performs two main independent motions: flexion-extension movements of the tibiofemoral joint within a sufficiently large range (up to 140°) in the в sagittal plane and limited rotational movements (up to 10°) of the shinbone in the horizontal plane at the terminal extension stage. When deflecting the knee through over 45°, the shin relaxes and the limiting functions of the capsule joint apparatus can increase sizably.
In addition to these complicated joints, other joints almost coincide with a sheave. The talocrural joint can be likened to two cylinders resembling a well-fitted radial bearing with a single degree of freedom practically and limited rotation limited by the articular apparatus. The joint between the shoulder and elbow bones has a similar structure serving just to bend and extend the forearm in the elbow. In this case, the extra lateral movement of bones is impossible because the collateral ligaments are close to the axis of rotation and the sliding surfaces of this sheave-like “pivot” have a specific anatomic structure.
This different natural design of the joints is certainly dictated by their specific locomotor functions and simultaneously the need to maintain high mechanical properties [5]. Therefore, from the viewpoint of the mechanics, the joints of animal and human extremities are very effective kinematic pairs capable to withstand considerable loads during prolonged period.
Investigation of the principles of the structure and functioning of different elements of the human and animal locomotorium revealed that both the position and the resulting forces affecting on partial elements of joints and neighboring bones [6, 7] determine their physiological loading in natural conditions. The resulting force pressing on the head of the hip joint is composed of the body weight and muscles that stabilize the pelvis normally in case the body center of gravity displaces (Fig. 1.2).
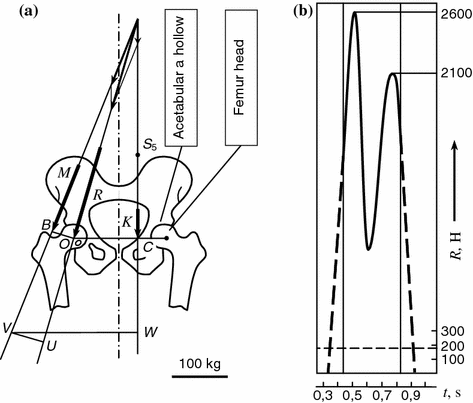
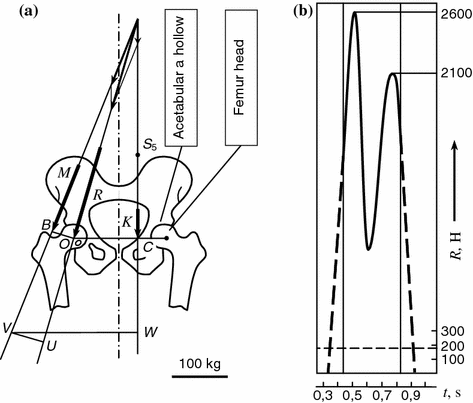
Fig. 1.2
Main elements of hip joint pivot unit a and diagram changes of compressive forces in hipbone head within step cycle b [8]. S 5 body center of gravity; K forces of action of body weight part; M forces of action of lateral abductor muscles; R resulting compressive forces
The line of the resulting effect passes medially across the hip head and laterally to it at a vertical angle 15–20°. As a result, the diagram of loading of the hip joint has the peak loadings acting on the hip head and exceeding the body weight three to nine times, i.e. the order of magnitude of 700–6300 N. These peaks appear at extreme amplitudes of oscillations, then the loading drops to the minimum in the sweep middle. Thus, the hip joint has to withstand during a year up to 2.5 million of cyclic loads and accomplish a considerable scope of movement, such as bending-unbending within a range of 45–60°, extension-contraction at the same time within a range of 11–12°, rotation through 6–14°, at a relative sliding speed of articular surfaces in different periods of a walking cycle 0.01–0.05 m/s.
The biomechanics of other elements of the human and animal locomotorium have its own specific features [8, 9]. The hip joint bends to almost 160° [10, 11], however, during normal ambulation the bending ranges just within 60–67° [12]. The shinbone in the unsupported phase is in the position of external rotation in respect to the hip condyle. At the moment the foot reaches support when the knee fully unbends, it acquires active and passive stabilization by the articular, capsular, and muscular apparatus. When the hip condyle reaches the terminal unbending stage the condyles rotate internally in respect to the tibial plateau.
Rotation of the shinbone is limited by collateral joints actively and by a specific configuration of the articular surfaces passively [1, 13]. The sections show that axial compression is capable independently to stabilize the unbent knee after transversing all the ligaments, including the cruciform ones. The cruciform joints limit actively the displacement of mated articular surfaces in motion in the ventrodorsal direction. The posterior cruciform joint is the main knee stabilizer. Relaxation of all the ligaments during rotation in the terminal unbending stage is a prerequisite of the normal functioning of the knee joint because it reduces the contact pressure on the medial portions of the shinbone. It is noteworthy than unbending of the knee increases the curvature of the hip condyles, normally more of the internal always than the external one.
Moreover, the essential role in the knee stability belongs to the capsular-articular elements and meniscuses , especially in the retromedial portion of the joint [14].
As the knee joint motion is exposed to five forces: axial, ventrodorsal in the sagittal plane, medial lateral in the horizontal plane and two moments of rotation relatively to the shinbone bone axis in the ventrodorsal axis, the ventrodorsal and medial lateral forces play just a secondary role as compensating forces to balance movements in these directions. At the same time, the forces of rotation in relation to the shinbone axis bone and ventrodorsal axis can redistribute the forces of flow from the lateral portion of the joint towards the medial portion. It is believed that the exterior hip condyle in the physiological conditions is loaded three or four times heavier than the interior condyle [14]. The knee joint may experience considerable forces that depend on the dynamic loading conditions. For example, it is noted in [15] that its elements when ascending a stairway may be affected by forces of the order of magnitude 150 Nm; when standing up after low crouching this joint can exposed to the loading five times exceeding the body weight.
Nevertheless, the time dependence of the resulting forces in the knee joint can have a similar pattern to that in the hip joint: it is determined by a significant straining of muscles and ligaments in addition to the load bearing force.
The latter play quite an important role in the knee joint biomechanics , controlling the physiologically comfortable positions, redistributing and reducing the compressive forces affecting the articular contact [8]. The paper [16] that changes of the length of ligaments redistribute contact forces, while shifts in the points of attachment of muscles do not practically affect them.
The effects in motion of the knee joint due to the spatial position of the centers of its rotation contribute sizably to the biomechanical process. It is known that the knee due to the ellipsoid form of the hip condyles does not have any permanent center of rotation typical for common pivots. Therefore, when it unbends and the radii of curvature of the condyles grow the center of rotation move along an arcuate line. However, the latter deviates from the line of arrangement of momentary centers of rotation of the joint. It can be traced vividly if one imagines the joint as a closed crossing four-link kinematic chain with two parts having the same degree of relative motion freedom, i.e. ignoring conventionally rotation of the shin bone in the terminal unbending stage and assuming that the cruciform joints are constantly stretched (Fig. 1.3). Modeling of rotation of links AD, BC (the cruciform joints) and AB (the tibial bone) around the fixed hip link DC enables to obtain a dorsal movement of momentary centers of rotation best reproducing the functional features of the natural knee joint. The diagram makes it ultimately clear how to interpret many features of functioning of joints in physiological norm and in pathology .
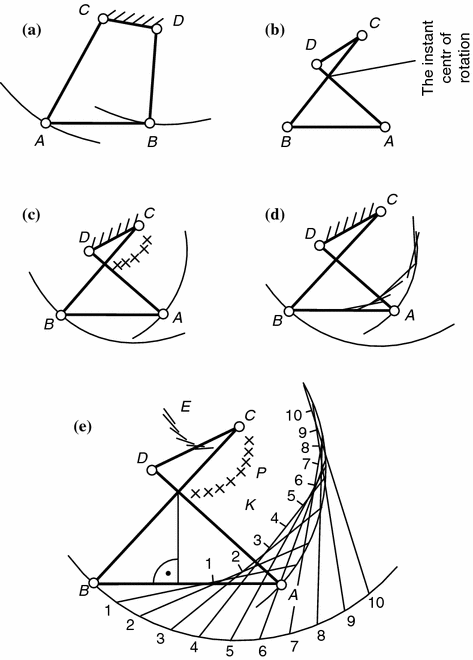
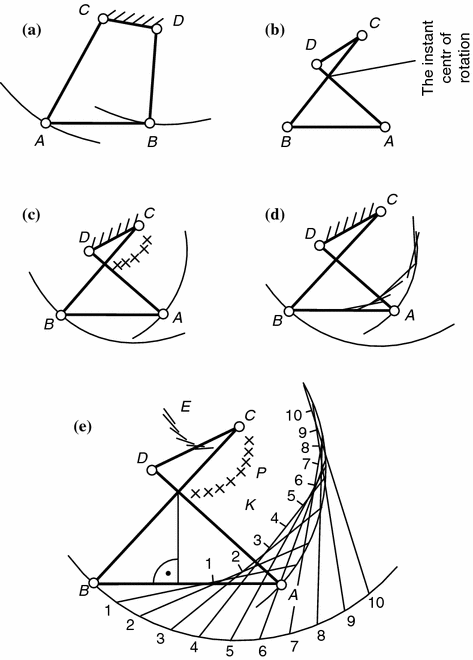
Fig. 1.3
Four-link knee joint kinematic chain: a closed Four-link kinematic chain; b crossed kinematic chain; c crossed kinematic chain showing passage of axis of motion of knee through momentary center of rotation in point of intersection of cruciform ligaments when hip component is fixed; d crossed kinematic chain showing curvature of contours of hip when shinbone moves; e crossed kinematic chain showing geometrical arrangement of tibiofemoral contact points during knee bending [13]. AB intercondyle shinbone hill; BC crossed kinematic chain of anterior cruciform joint; AD posterior cruciform joint
The analysis in [17] proves that application of a longitudinal compressive force via a joint to a joint boosts its stability, on the one hand, but limits its degrees of freedom and that can change the joint mechanical properties due to movement of the momentary centers of rotation in space. A position of the knee joint center of rotation depends on the extent of initial compression and can displace by over 0.02 m. An essential fact too that the momentary centers of rotation in the hip intercondyle pit (the fixed element) of the lie mutually closer than in the unloaded joint. As a result, the sliding speeds under these conditions differ little and their directions coincide with the articulation slot line. A significant scatter of the momentary centers of rotation, e.g., in case of injury of the meniscus, ligaments, or changes in the configuration of mating surfaces, induce extra forces with the speed vector different from the articulation slot line and directs it a certain angle to it. Friction in the joint intensifies joint, the cartilage is continuously microtraumatized, degeneration centers appear, and mechanodestruction follows.
Therefore, operative interventions are biomechanically justified when it is necessary to correct the centers of rotation of pathologically altered joints and can restore their normal functioning in a number of cases.
From the viewpoint of transmission of the supporting loading the geometrical characteristics of joints are essential, such as forms of dynamically contacting surfaces and their mutual compliance (congruence) that determine the extent of mobility of articular ends, the amplitude of their motion and the number of axis around which they move. It is believed that working contact joints are fully loaded in only one position corresponding to transmission of the maximum force via the joint [18]. The articular contacts in other positions are incompatible, i.e. incongruent. As a result, loads of various magnitudes and directions in the hip joint can produce four contact types: a usual contact that occurs under inconsiderable loads; a contact in response to a position of joints; a contact in response to loading and occurring only under considerable loads; no contact between surfaces at all at any joint position and load.
The data about the actual contact area in different joints are controversial. Paper assumes that the actual contact area in each knee joint condyle under load is 1–2 cm2. However the results in [12] show that the mean supporting area of the interior condyle of the unbent knee is 2.5–6.7 cm2, that of the exterior condyle is 1.7–5.1 cm2, i.e. 1.6 times less than that of the interior one. It is believed that the surface contact reaches 4 cm2 in the hip joint under full loading. Thus, it is apparent that the articular surfaces have sufficiently high contact pressures of 60–70 kg/cm2 under maximum loads exceeding the body weight three to nine times [19].
Mechanical interactions between articular surfaces are known to occur always through the layer of a compliant composite material—the hyaline cartilage of irregular thickness. The cartilage layer gradually thins away in contact zones in the direction towards the edges of articular surfaces. It is probable that the geometry of the articular cartilage in the contact zone that affect considerably the mechanism of transmission of mechanical forces in the joint. In fact, analysis of the contact interaction of one solid body with another through the elastic interlayer from a homogenous material shows that the geometric characteristics of the interlayer strongly affect both the specific pressure and the pattern of its distribution (Fig. 1.4).
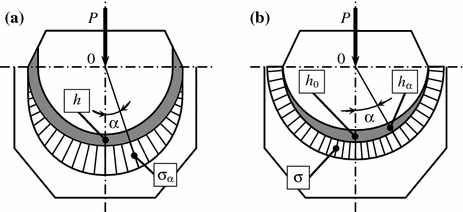
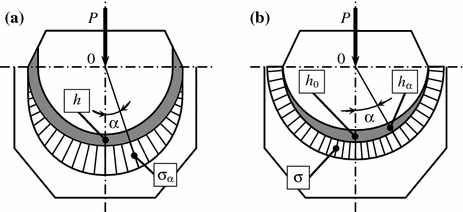
Fig. 1.4
Diagram of contact interaction between two solid bodies separated by an elastic interlayer of inhomogeneous material in response to angle of contact α: a specific pressure sinusoidal distribution; b regular specific pressure distribution
Constant thickness of the elastic interlayer changes the specific pressure in response to the contact angle according to the sinusoidal law, meanwhile the thickness of the elastic layer is variable according to the law of mass and the specific pressure distribution in the contact region is regular. The fact that the cartilage layer in the contact zone between articular surfaces narrows towards the edges permits to assume a similar pattern of distribution of contact forces in joints. Hence, the thickness distribution of the cartilage in the joint is such that during ambulation the pressure on the articular surfaces distributes regularly in the contact region. Whence an essential conclusion is that the cartilaginous layer is a peculiar shock absorber of dynamic loads favoring regular distribution of pressure in the joint. Table 1.1 shows examples of the moduli of elasticity of some materials most frequently used for prosthetic arthroplasty.
Material | Modulus of elasticity × 103, MPa |
---|---|
Joint cartilage | 0.001–0.17 |
Natural rubber | 0.0025–0.1 |
Silicon rubber | 0.01 |
Super high-molecular polyethylene | 0.5 |
Bone cement | 3.0 |
Bone | 10.0–30.0 |
Alloy Ti–Al–Va | 106.0 |
Stainless steel | 205.0 |
Alloy Co–Cr–Mo | 230.0 |
Aluminium | 350.0 |
Table 1.1 shows clearly that just few possess the mechanical properties comparable with the properties of the joint cartilage as a natural composite material.
Some human and animal joints have fibrous cartilaginous structures or the interarticular cartilage known as the meniscus in addition to the hyaline cartilage coating the bone [1, 2]. For example, there is a medial meniscus and a lateral meniscus in the human knee joint. In the joint they balance the mismatch between the hip condyle and the shinbone, expand the actual contact area. Their configuration demonstrates it (Fig. 1.5).
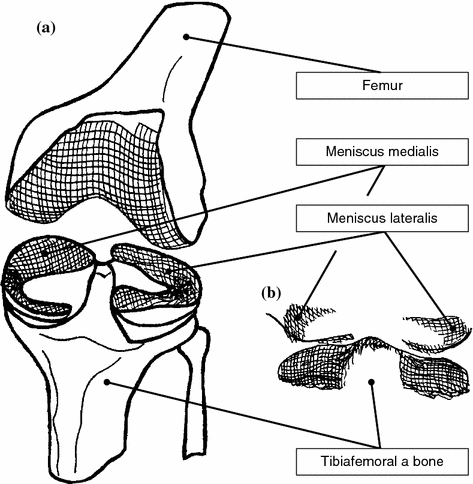
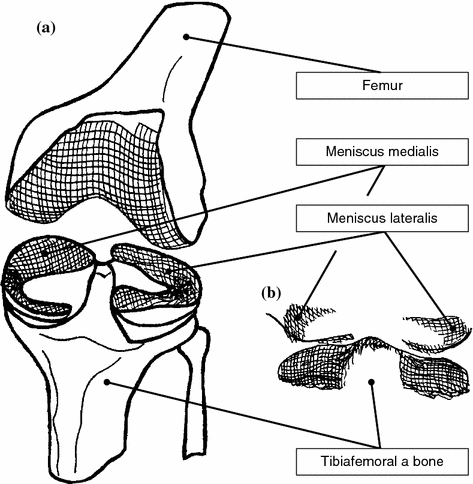
Fig. 1.5
Diagram illustrating contact interaction in knee joint, (a) and stereogram representing contact zones between hip condyles, tibial plateaus and meniscus, (b)
The peripheral part of each meniscus is thicker and convex; the central part has a fine free edge. The sagittal section shows an edge-like shape. The medial meniscus resembles a hemisphere that arranges laterally over the entire circumference of the respective shinbone condyle . As a result, the external edges of the meniscus repeat the configuration of the external edges of the tibial plateaus. It is believed that a high congruence of articular surfaces provided by meniscuses strongly contributes to the joint stability, even if anterior cruciform joint is injured [20, 21].
The unique dumping properties of the cartilaginous tissue in the meniscus, their good adaptability to articular surfaces and extensive contact area with hip condyles play an essential role in undertaking and redistributing continuously variable specific pressure in the joint [1]. According to [20], the meniscus bears up to 50 % of the compressive loading when the knee is unbent and up to 85 % when the knee is 90° bent. It is significantly because that the meniscus is capable to change its configuration in response to the angle of bending of the knee joint assisted by the joint capsule along the edges of the tibial plateaus that arrest the meniscus peripherally resisting the effect of the forcing out forces appearing under rather heavy axial loads [1].
A total or subtotal removal of the internal meniscus shrinks the contact in the medial portion of the joint by 50–70 %, hence, the specific pressure on jumps sharply [22]. It may lead to gradual impairment of the dumping properties, degeneration, and mechanical destruction of the cartilage. Numerous clinical observations confirm this fact [23]. A number of experimental models of the early osteoarthrosis are based on the meniscus resection [21, 24]. It is noted that degenerative changes in the joint are pronounced and proportional to the area of the removed meniscus segment [20, 22]. That is why in recent years the concept of partial or so-called intermeniscus resection is gaining recognition in case meniscus damage; the progress of the concept is due to adoption of modern arthroscopic medical surgical equipment [25].
The experiments have shown that partial meniscus ectomy leads in the majority of cases to a significant dysfunction of joints [25]. A probable explanation of this behavior of the locomotorium is that, if the cartilage is normal, and just a slight of the affected meniscus is removed, distribution of stresses in the joint remains practically unchanged and regular [1]. However, it is possible in such cases that arthrosis may reappear [26]. One of the causes of its development may be a disorder of the congruence of the articular surfaces after an operative intervention. It can produce zones the contact of cartilages is fully dependent of the loading magnitude and occurs only in case the forces are large and the zone also, where there is no contact at all under any load at any position of the joints. This boundary region between the zone of permanent contact and its absence is the most sensitive and vulnerable to appearance and development of really centers of degenerative changes in the cartilage. Other more detailed studies confirm it too [1, 22, 23].
Thus, the accomplished analysis of joints as versatile kinematic pairs capable to withstand significant variable loads shows that, alongside with their features, structure, functioning, and biomechanics, the articular cartilage plays an essential role in transmission of mechanical interactions between mated bone surfaces. To perform this function, the cartilaginous tissue should possess specific biomechanical properties that differ from those of other tissues [27]. Their unique structural arrangement in the cartilage can explain it.
1.2 Structure and Functions of Joint Cartilage
The first works in the sphere of chemical composition and structural organization of the cartilage matrix relate to the mid of the last century when J. Hunter studied the morphology of a pathologically altered cartilage in vivo. In 1837, E. Müller exposed the cartilage to heavy pressure and obtained a solution of a substance that he called chondrine [28]. Later K. Krukenberg separated the main chondrine component and identified it as chondroitin sulfate. In 1953 E. Davidson, K. Meyer, A. Linker and B. Weissmann discovered one more substance that they believed initially a constituent cartilage component and called keratan sulfate. In these years K. Meyer with his disciples showed that chondroitin sulfates were a variety of polysaccharides; their molecules were chains of disaccharide links alternating with the glucuronic acid and N–acetylgalactosamine—a sulfate derivative (Fig. 1.6a, b). Later it was established that keratan sulfate is the same polysaccharide, but it consisted of alternating molecules of galactose and the sulfate derivative N–acetylglucosamine (Fig. 1.6c).
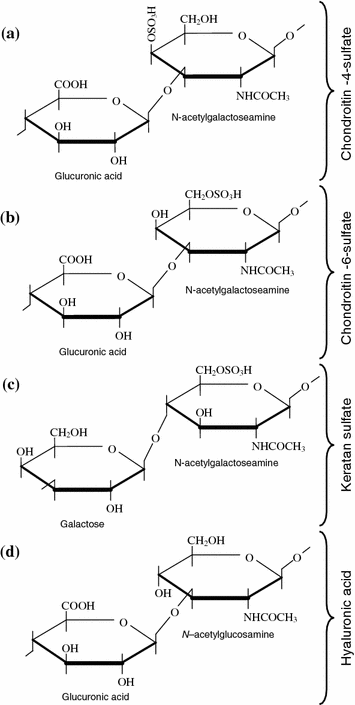
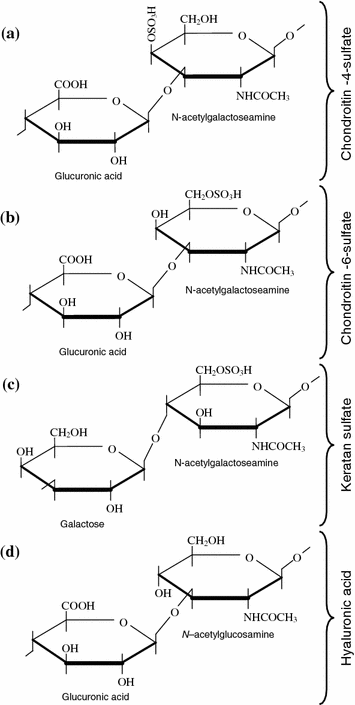
Fig. 1.6
Chemical structure of glucose aminoglycans in the joint cartilage
Though certain results were achieved by the studies of the chemical composition of the constituent components of the cartilaginous tissue, the first full idea about its structure appeared only in 1969 when the methods used to study of nucleic acids were successfully applied to extraction of undamaged giant aggregates of macromolecules of proteoglycans from the cartilage [27, 28]. In the same year, an assumption was made that the structure protein polysaccharide complexes in the cartilage matrix was not limited to appearance of the aggregates of proteoglycans, that there existed larger complexes contributing to stabilization of the structural organization of the joint cartilage and maintenance of its biomechanical properties. In 1983 this assumption was confirmed in [29] showing that the aggregates of proteoglycans were combined by two special glycoproteins from the cartilage matrix into still larger structures or superaggregates.
Thus, so numerous biochemical experiments, application of electron microscopy enabled to produce a structural characteristic and to visualize the protein polysaccharide complexes and to get general ideas about the fine structure of the proteoglycans and their aggregates (Fig. 1.7) [27, 30].
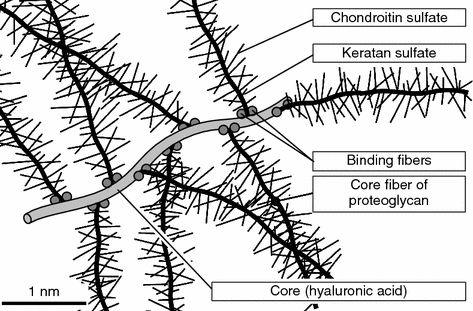
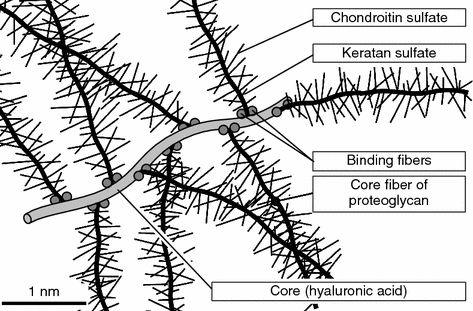
Fig. 1.7
Fragment of aggregate of proteoglycans. Proteoglycans (subunits) are attached in a non-covalent manner with two special binding proteins to hyaluronic acid molecule [30]
The latter account for 50 % of the dry weight of the joint cartilage and represent giant macromolecules that include the hyaluronic acid (HUA), Glycoproteins and stem or medullar proteins with numerous lateral chains of polysaccharides (glucose aminoglycans) play an essential role in their composition. At present these ideas are generally accepted because they provide fuller explanation of the functions of proteoglycans in creating the physico-chemical and biomechanical properties of cartilaginous tissue, though some details are continuously verified and added [28].
According to the generally accepted ideas, the main proteoglycan aggregate in the e cartilage matrix is the HUA molecule (Fig. 1.7). It belong to unsulfated glucose aminoglycans and consists of alternating remnants of the D-glucuronic acid and N-acetylglucosamine (Fig. 1.6d). Compared with other glucose aminoglycans and the HUA it has a larger molecular weight and can combine up to 200 proteoglycan subunits with their points of joining being spaced by at least 50–60 disaccharide links [27]. The averaged composition of a proteoglycan subunit (a macromolecule) is shown in Table 1.2. Since practically the entire HUA molecule in the cartilage matrix is involved in production of proteoglycan aggregates , it determines their sizes.
Components | Number of chains | Molecular mass | Part of total macromolecular mass, % |
---|---|---|---|
Protein | 1 | 200000–300000 | 7–12 |
Chondroitin sulfates | 100 | 20000 | 80–85 |
Keratan sulfate | 50 | 5500 | 7 |
Oligosaccharides | 50 | 1200–2000 | 1–3 |
Charged groups | |||
Sulfate | 4500 | ||
Carboxylate | 4200 |
Polypeptide chains of medullar proteins branch away from the HUA like from a stem and form the base of the proteoglycan subunits (Fig. 1.7). The proteoglycan subunits attach to the HUA in a non-covalent manner with a specific terminal region having a globular conformation [31]. Moreover, the so-called combining proteins typical only for the joint cartilage matrix [32, 33] additionally stabilize these portions of the proteoglycan aggregates. A multitude of polysaccharide chains attach, in their turn, to each long medullar protein molecule [28]. They are chiefly sulfated varieties of glucose aminoglycans: chondroitine-4-sulfate, chondroitine-6-sulfate, and keratan sulfate (Fig. 1.6).
Both chondroitin sulfates consist of alternating remnants of the glucuronic acid and galactose amine and are alternating copolymers of the D-glucuronic acid and N-acetylgalactosamine. The O-sulfate group attaches to them and localizes in the molecule determining the difference between these isomers having a practically identical structure. The length of the chains of the chondroitin sulfates in the cartilaginous tissue is made up by 30–60 disaccharides on the average [1]. Quite often, the molecules have a hybrid structure when the same chain has disaccharide units , such as both condroitine-4- and condroitine-6-sulfate [27]. However, condroitine-6-sulfate dominates in the cartilage matrix.
Keratan sulfate is the only glucose aminoglycans free of hexauronic acid. Its molecule is a polymer composed of a repeating disaccharide unit of N-acetylglucosamine containing a sulfate group and D-galactose near the sixth carbon atom [27]. The keratan sulfate chain is somewhat shorter than the chondroitin sulfate chain and usually it is about 15–30 disaccharide units.
All glicoaminoglycans in the cartilage matrix have a significant number of anion groups. Each disaccharide link, e.g., in the chondroitin sulfates one the saccharides, vis. the glucuronic acid, contains a carboxyl group (COOH–), while another is an N-acetylgalactoamine-sulfatene group (SO4 2–). Therefore, the polyanionic pattern of glucose aminoglycans is the main factor governing their physico-chemical and biomechanical properties. Due to this polyanionic nature and saturation with free acid (carboxyl and sulfatene) groups with similarly (negatively) charged glucose aminoglycans, they are straightened in the proteoglycan subunit and mutually repel. Hence, they are oriented perpendicularly to the medullar proteoglycan subunit in the shape of a peculiar wire brush (Fig. 1.7). Due to the same electrostatic interactions still, the proteoglycan monomers, in their turn, form an analogous structure of proteoglycan macromolecules arranging perpendicularly to the HUA chain.
Because of such arrangement features, the fine structure of proteoglycans and their aggregates acquire the properties of the so-called diffuse macromolecules because voids appear in the intervals between their glucose aminoglycans units that act like a sponge and can selectively accumulate low- molecular components, such as water molecules and other comparatively fine ingredients of the proteinopolysaccharide complex. Therefore, the proteoglycans in the joint cartilage matrix possess a huge structuring capability concentrating a significant quantity of water whose mass exceed much it own mass. Hence, the articular cartilage is a highly hydrated with water being one of its main components.
The ratio between proteoglycans and water determines the degree of its possible loss by the cartilage, i.e. the higher the concentration of proteoglycans the less water can leave the cartilage under the effect of contact pressure in locomotion, and vice versa, lack of proteoglycans due to reduction of the concentration of glucose aminoglycans, e.g., in osteoarthrosis, leads inevitably to the softening of the cartilage matrix and its stronger permeability followed by development of pathological changes [34, 35].
The osmotic pressure due to the protein polysaccharide complexes is known to be responsible for the ability of the cartilage to withstand high mechanical pressure without losing water and for the fluid balance. Until quite recently, it was believed that the concentration of free water in the cartilage matrix was about 94 %. However, more recent studies give different values of interstitial water concentration in cartilages—70–100 %, it is apparently due to concentrations of proteoglycans in them [27].
This free behavior of the interstitial water and its compressibility add essential biomechanical properties to the articular cartilage; their sense is the following. When the cartilage experiences pressure, water is forced out from the regions of sulfate and carboxyl groups where the charge arrested them. At the same time these groups converge and the forces of repulsion between their negative charges inhibits further compression of the tissue. When the compression disappears, water returns and makes deformation under load reversible [36]. Hence, the interstitial water together with the proteinopolysaccharide complex with its inherently high osmotic pressureм and huge structuring ability due to the high density of the negatively charged group of sulfonated glucose aminoglycans maintain the elasticity and stiffness of the joint cartilage, i.e. it is responsible for the shock-absorbing properties in joints.
Apparently, the high strength properties of the cartilaginous tissue would be impossible without its highly ordered supermolecular organization. The intercellular matrix or collagen as the third component plays an essential role. It represents specific proteinic macromolecules or their aggregates forming the connective tissue in the fibrous structure.
At present, it is believed that there are up to 25 types of collagens [1, 35, 37]. The structural or fibril forming collagens (types I–III) are the main in the connective tissue. They typically perform mechanical functions. It is known that the collagen type I dominates in bones, skin, tendons, joints, and meniscus. The collagen type III dominates in muscles, blood vessels, visceral organs [1, 36–38]. The collagen type II dominates in the articular cartilage. Its concentration in the intercellular matrix is 20–25 % exceeding the concentration of proteoglycans (5–10 %) and ranks second after water (65–80 %).
All structural collagens possess high mechanical properties (Table 1.3) [39].
Material | Young modulus, MPa | Breaking strength, MPa |
---|---|---|
Collagen | 103 | 50–100 |
Elastin | 0.6 | – |
Bone | 104 | 102 |
Cured rubber | 1.4 | – |
Oak | 104 | 102 |
Mild steel | 2·105 | 5·102 |
Their fivers are capable to stretch 10–20 % without rupturing. Transverse glycoprotein interfibrillar links have an essential significance in the mechanical properties of the structural collagens. They prevent mutual sliding of collagenous fibers , water presence is also essential [1]. It typical for water to change the protein density without affects its shear modulus.
The methods of X-ray structural analysis and electron microscopy revealed that portion of regular transverse striation repeat periodically after every 60–70 nm of the longitudinal fibrous collagenous structure. There are grounds to assume that it is due alternation of highly ordered crystalline portions of fibrils with zones having aminoacidic composition and less regular arrangement of molecules. The collagen in fibrils probably stretches predominantly [39].
To understand the role of the collagen individually in maintaining the main biomechanical functions of the cartilage integrally the data known at present about its molecular and supermolecular structural organization are essential. Their essence is the following (Fig. 1.8).
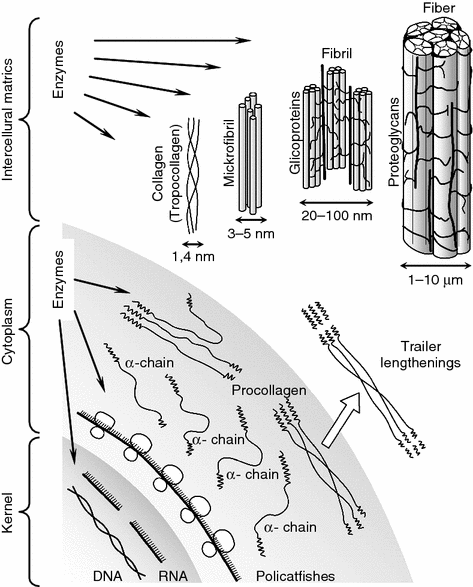
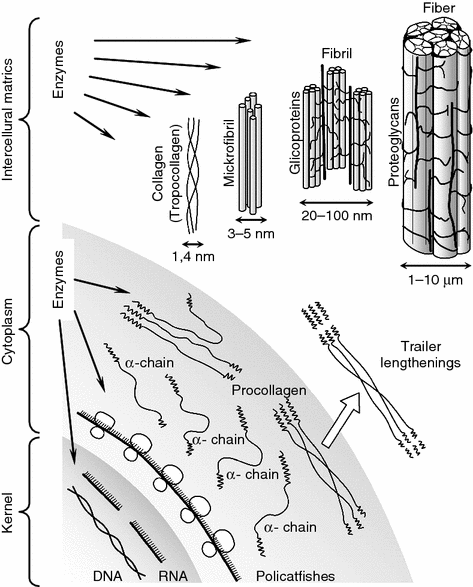
Fig. 1.8
Diagram биo synthesis collagen and forms of collagenous structures
The mature collagen in the connective tissue is preceded by procollagen macromolecules that are its intracellular form consisting of left-handed α-chains. Both covalent hydrogen bonds combine the latter and into an extended right-handed spiral. Terminal extensions split from the procollagen macromolecules in the extracellular space, this results in tropocollagen macromolecules 300 nm long and 1.4 nm in diameter. They combine by cross-intermolecular covalent links and form microfibrils 3–5 nm in diameter. The following stage is that several microfibrils form collagenous fibrils 20–100 nm in diameter with the participation of polysaccharide components—proteoglycans and glycoproteins. There are intermolecular transverse (cross) links in the portions of adjacent macromolecules of the fibrils. The integrity of these intra- and intermolecular cross-links together wit hydrogen bonds stabilizing the spiral configuration of α-chains maintains the high structural and mechanical strength of the collagenous fibers.
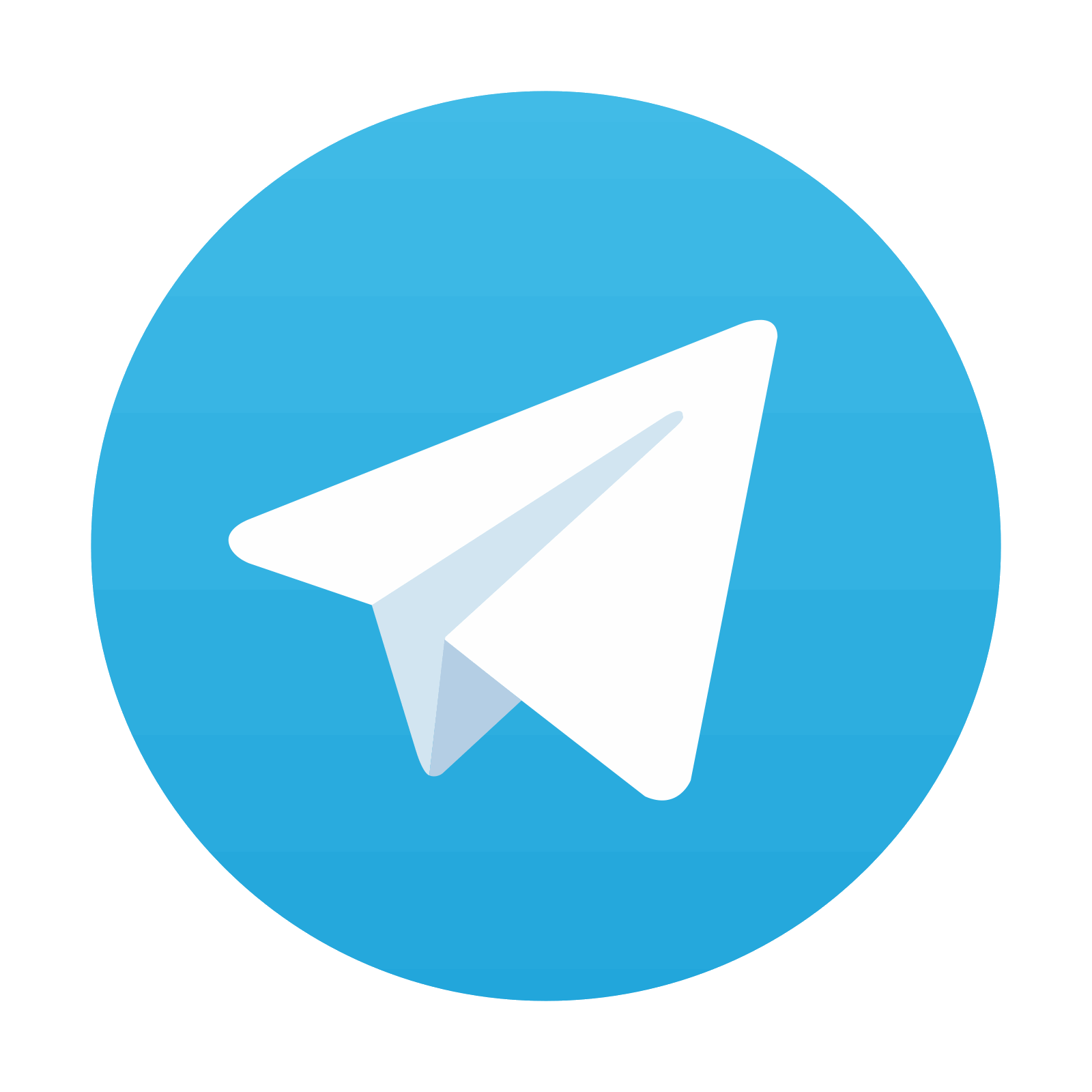
Stay updated, free articles. Join our Telegram channel
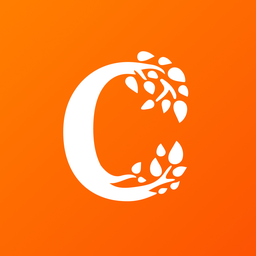
Full access? Get Clinical Tree
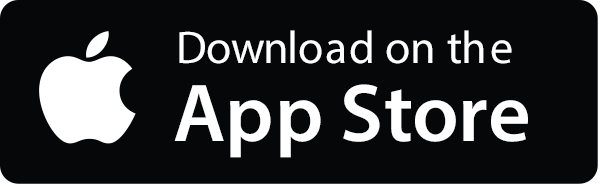
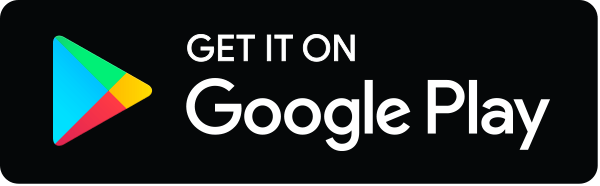