11 Xintong Wang1, Angela L. Zachman1, Simon Maltais2, and Hak-Joon Sung3 1Department of Biomedical Engineering, Vanderbilt University, USA 2Department of Cardiac Surgery, Vanderbilt University, USA 3Department of Biomedical Engineering, School of Medicine, Vanderbilt University, USA Stem cells and progenitor cells reside in a complex microenvironment consisting of multiple types of extracellular matrix (ECM) components, including matrix proteins, proteoglycans, hyaluronic acid, and growth factors deposited on or tethered to ECM. These ECM components present a great variety of ligands and provide mechanical and topographical cues to stem cells. The extracellular cues are sensed by stem cells and trigger intracellular signaling pathways, thereby determining the self-renewal or differentiation of the stem cells. Working closely with surrounding cells through direct cell–cell contact or via paracrine pathways, these extracellular cues form “niches” and help to define stem-cell fate. As an important field of regenerative medicine, tissue engineering is becoming increasingly attractive with the fast-paced development of biomaterials. Stem cells are in a unique position to satisfy the needs of regenerative medicine due to their potential to differentiate into multiple lineages. The tremendous progress in stem-cell research provides greater opportunities to regenerate tissues and organs than we could ever imagine. In addition to using biomaterial scaffolds to improve the growth and proliferation of fully differentiated somatic cells, stem cell-based regenerative medicine requires the scaffolds to support and control the differentiation of stem cells. Therefore, understanding the interactions between stem cells and biomaterials (“structure–function relationships”) is critical to the design and fabrication of functional products. On the other hand, elucidating how ECM ligands and the physical properties of substrates regulate stem-cell fate will provide insight into the development of new biomaterials for successful tissue regeneration. In this chapter, we introduce the roles of ECM-derived biomaterials in regulating stem-cell differentiation, which include Matrigel, collagen, laminin, and fibrin, followed by the applications of typical non-ECM-derived natural and synthetic biomaterials in the control of stem-cell fate. The progress of this area within the past 10 years is discussed. We aim to provide a comprehensive but concise review of the biomaterials being applied to direct stem-cell differentiation for regenerative medicine. Natural biomaterials, including both ECM- and non-ECM-derived components, have been used to produce scaffolds for tissue regeneration. Many ECM components, such as collagen, fibronectin, hyaluronic acid, proteoglycans, and hydroxyapatite (HA), are widely applied. Non-ECM components, such as chitosan, alginate, and silk, have also been used to fabricate scaffolds. The advantage of using these natural materials is that they mimic the physiological environment that stem cells encounter in vivo by providing ligands similar to those found in the stem cell niche. However, the disadvantages of using natural materials in regenerative medicine include: (i) the difficulty in controlling the chemical and physical properties of the material for different applications; (ii) batch-to-batch variation; (iii) the possibility of pathogen contamination; and (iv) in vivo immunogenicity with materials of animal origin. In spite of these drawbacks, natural materials are still being extensively used in tissue engineering due to their similarity to the stem cell niche and the ease with which they can be obtained from commercial sources, with minimal manipulation needed before application. Matrigel is a superior substrate with which to maintain and differentiate stem cells. It is derived from murine sarcoma cells and consists of type IV collagen, laminin, a small amount of heparan sulfate proteoglycan, and growth factors [1]. It is commercialized by BD Biosciences. Matrigel has been used as a feeder-free substrate for a wide selection of stem cells, especially the pluripotent stem cells, such as embryonic stem cells (ESCs) and induced pluripotent stem cells (iPSCs) [2–19]. It has also been used in the differentiation of pluripotent stem cells to all three germ lines. For example, mouse embryonic stem cells (mESCs) were grown on Matrigel and were differentiated to thyroid follicular cells [18]; pancreatic insulin-producing cells were obtained from ESC-derived endoderms on Matrigel [3, 8]; hepatic cells were differentiated from ESCs that were encapsulated in Matrigel and loaded into a poly(lactic-co-glycolic acid) (PLGA) scaffold [10]. Interestingly, Matrigel and type I collagen supported the hepatic differentiation of human embryonic stem cells (hESCs), but hESCs could not attach to laminin, even though laminin is one of the major components of Matrigel [12]. The growth factor-reduced Matrigel efficiently supported survival and neurite outgrowth of neural precursor cells in vitro, unlike collagen and laminin. It also promoted the proliferation and differentiation of neural precursor cells in vivo, resulting in an increased number of neurons through suppression of the inflammatory response [20]. Besides supporting pluripotent stem cells to differentiate along the endoderm direction, Matrigel also aids in the differentiation of pluripotent cells to mesodermal lineages, such as vascular [4, 9, 14, 16], cardiac [5, 19], and mesenchymal stromal cells [21]. Matrigel has traditionally been used as a substrate to demonstrate the vascular-tube formation of stem cells. Moreover, Nakagami et al. have reported that sprouting blood vessels could be differentiated from murine ESC-derived embryoid bodies (EBs) on Matrigel, but not on collagen or gelatin [16]. Remarkably, a Matrigel sandwich method was developed to differentiate human induced pluripotent stem cells (hiPSCs) to cardiomyocytes. By seeding iPSCs on Matrigel and subsequently overlaying another layer of Matrigel on top, this method promoted epithelial–mesenchymal transition (EMT) and substantially increased the differentiation of iPSCs to cardiomyocytes in vitro with high efficiency (∼98%). A pool of mixed cardiomyocytes, including embryonic nodal, atrial, and ventricular cardiomycoytes, was obtained after 30 days [19]. Although Matrigel is a wonderful substrate for supporting the growth and differentiation of many types of stem cell, its complex and heterogeneous composition makes isolating the effect of individual components very challenging, indicating that biomaterials with a simple composition are required to better predict the stem-cell fate. Fibrin gels or fibrin networks are created during the natural wound-healing process when fibrinogen is converted to fibrin by thrombin and the fibrin strands are then crosslinked in the presence of factor XIIIa. Thus, fibrin is considered another type of natural material that can be applied to support stem-cell differentiation. The effects of fibrin on endothelial progenitor cell (EPC) growth and differentiation were studied at different fibrinogen–thrombin ratios. The viability, differentiation, and angiogenic capabilities of EPCs were evaluated and compared to EPCs grown on fibronectin. Fibrin formed a porous network in vitro, and the stiffness significantly influenced the growth of EPCs. Cell viability was found to be higher on fibrin than on fibronectin. EPCs on fibrin differentiated to vascular cells and released cytokines to recruit other cells, indicating that fibrin could serve as a suitable matrix for EPC growth, differentiation, and angiogenesis [22]. In another study, fibrin was mixed with fibronectin, gelatin, and growth factors, which successfully induced the differentiation of EPCs and smooth muscle progenitor cells to endothelial and smooth muscle cells (SMCs), respectively [23]. Fibrin gels can be used in bone regeneration. Osteogenic differentiation of human mesenchymal stem cells (hMSC) seeded in a fibrin sealant was analyzed by altering the fibrin gel composition. Cell proliferation was higher with a low concentration of fibrinogen, but when the fibrinogen concentration was increased the alkaline phosphatease (ALP) activity, bone sialoprotein gene expression, and small nodules of mineralization were enhanced after 3 weeks. However, there was no significant increase in osteocalcin expression after 4 weeks. These results suggest the osteogenic differentiation of hMSCs is dependent on the fibrinogen–thrombin ratio of the fibrin sealant, but hMSCs did not fully differentiate to mature osteoblasts [24]. Autologous platelet-rich fibrin glue containing canine bone marrow-derived mesenchymal stem cells (BM-MSCs) was used to regenerate bone defects with or without MEDPOR. Radiographic analysis 4 months post-transplantation showed an average of 72.8 ± 8.02% new bone formation with MSC-containing fibrin glue guided by MEDPOR and a 53.34 ± 6.87% new bone formation in the group with MSC-containing fibrin glue without MEDPOR, compared to 15.14 ± 2.37% in a control group with fibrin gel alone. Histological analysis demonstrated the defect was repaired by typical bone tissue in MSC-containing fibrin glue both with and without MEDPOR, whereas only minimal bone formation was observed in the control group, showing the critical role of fibrin in supporting osteogenesis by MSCs [25]. Growth factor-loaded particles can be mixed in fibrin scaffolds to direct osteogenic differentiation via controlled release of growth factors. For example, a system combining fibrin and bone morphogenic protein 2 (BMP2)-loaded nanocarriers was applied for the osteogenic differentiation of hMSCs. The controlled release of BMP2 by nanocarriers significantly promoted the osteogenic differentiation of hMSCs embedded in fibrin scaffolds as compared to non-BMP2 scaffold or BMP2-loaded fibrin constructs without nanoparticles [26]. Porcine MSCs were able to differentiate into adipogenic, osteogenic, and chondrogenic lineages when cultured on blood-derived autologous fibrin scaffolds [27]. Interestingly, fibrin microthreads were developed to embed hMSCs, and these could be used as a suture to enhance the efficiency and localization of stem-cell delivery. The cells were viable and proliferative in microthreads. Moreover, the hMSCs in the microthreads retained their multipotency and could differentiate to adipocytes and osteocytes, providing a potential platform for the efficient delivery of stem cells in vivo [28]. Fibrin scaffolds have also been applied in neurogenesis of stem cells. A fibrin scaffold was produced to release vascular endothelial growth factor (VEGF) in order to control the migration of murine neural stem cells (NSCs) (C17.2) for neuroregeneration. The stem cells embedded in collagen hydrogels were printed close to the VEGF-releasing fibrin gel. The C17.2 cells printed within 1 mm of the border of fibrin gel changed their morphology in the presence of VEGF and migrated toward the fibrin gel for a total distance of 102.4±76.1 μm over 3 days [29]. Other growth factors were also incorporated in fibrin scaffolds to direct the differentiation of ESC-derived neural progenitor cells. Examples include neurotrophin 3 (NT3), Sonic hedgehog (SHH), and platelet-derived growth factor (PDGF). Mouse EBs containing neural progenitors were seeded in these fibrin scaffolds. The simultaneous delivery of NT3 and PDGF from fibrin increased the fraction of neural progenitors, neurons, and oligodendrocytes and decreased the fraction of astrocytes compared to EBs in fibrin scaffolds without growth factors [30]. As the most abundant ECM protein, collagen favors stem-cell commitment toward many lineages. Its excellent and adjustable mechanical properties make it ideal for directing the differentiation of stem cells to myogenic lineages. The effects of collagen I and IV on mouse BM-MSC differentiation to SMCs were evaluated [31,32]. BM-MSCs cultured on collagen I and IV reduced the expression of SMC markers such as smooth muscle actin (SMA) and myosin heavy chain (MHC) compared to cells on tissue culture plastic. Growth factors including transforming growth factor β1 (TGFβ1) and PDGF-BB enhanced SMC differentiation on collagen. These results suggest that, unlike plastic, collagen tends to maintain the undifferentiated state of BM-MSCs in the absence of exogenous growth factors [31]. Human BM-MSCs seeded on collagen-coated silicon membranes underwent myogenic differentiation with the addition of insulin-like growth factor 1 (IGF1). The combination of mechanical loading and growth-factor treatment led to enhanced expression of myogenic markers such as Myf5, MyoD, MyoG, and Myf6, indicating the supportive role of collagen in myogenesis of MSCs [33]. Type I collagen was micropatterned on a polyacrylamide gel with a stiffness of 10.2 kPa, which efficiently modulated elongated focal adhesion complexes of hMSCs, and in turn preferentially recruited the β3 integrin cluster, regulated RhoA downstream signaling, and induced specific myogenic differentiation at both transcriptional and translational levels [34]. To mimic the stem cell niche of collagen-rich tissues such as cartilage and bone, collagen has been combined with other structural biomaterials and growth factors to direct stem-cell fate toward chondrogenic and osteogenic lineages. Human umbilical cord-derived MSCs (UC-MSCs) seeded in collagen hydrogels differentiated to chondrogenic cells after exposure to chondrogenic factors, as characterized by increased expression of collagen II, aggrecan, cartilage oligomeric matrix protein, and SOX9. Thus collagen hydrogels provide an appropriate 3D environment for the promotion of chondrogenic differentiation of UC-MSCs for cartilage regeneration [35]. In another study, when UC-MSCs were grown on poly(ϵ-caprolactone) (PCL)/collagen nanoscaffolds for 3 weeks cell proliferation and chondrogenesis were found to be higher than in human BM-MSCs. The authors also demonstrated that basic fibroblast growth factor (bFGF) in culture medium played a crucial role in chondrogenesis [36]. Other factors, such as mechanical stimulation and oxygen tension, were revealed to be important in directing the tenogenic lineage commitment of adipose tissue-derived stem cells on type I collagen gel [37]. Collagen-glycosaminoglycan (chondroitin sulfate or hyaluronic acid) scaffolds were applied to assay the effects of stiffness and composition on MSC differentiation without the addition of differentiation supplements. The scaffolds with lower stiffness (0.5 kPa) favored the chondrogenic differentiation of MSCs, while scaffolds with higher stiffness (1.5 kPa) directed cells to osteogenic differentiation, as revealed by the expression of SOX9 and RUNX2, respectively. Besides scaffold stiffness, the composition also influenced MSC differentiation. Upregulation of SOX9 and RUNX2 were observed with collagen-hyaluronic acid and collagen-chondroitin sulfate scaffolds, respectively, indicating hyaluronic acid is more efficient in chondrogenic differentiation and chondroitin sulfate is more potent in inducing osteogenesis. More importantly, these results demonstrate that the differentiation of MSCs can be controlled by intrinsic properties of the substrate in the absence of differentiation supplements [38]. Other than stiffness and composition, 3D collagen hydrogels were able to support the differentiation of MSCs to osteoblasts with osteoinductive supplements. Osteocalcin expression and calcium deposition were significantly higher in 3D hydrogel than in 2D culture [39]. The morphology of osteoblasts differentiated from MSCs in 3D collagen cultures was similar to that of osteoblasts in vivo, as compared to cells in 2D collagen cultures [40]. Matrigel has been shown to promote cardiogenesis by many groups. As one of the major components of Matrigel, collagen IV may be able to support cardiac differentiation of stem cells. In one study, collagen IV was applied in directing mouse ESCs to cardiac cells. It was demonstrated the fraction of Flk+ cells was higher on collagen IV than on fibronectin, laminin, or vitronectin in 2D culture. However, vitronectin induced the most Flk+ cells among all these substrates when tested in 3D culture system [41]. The result was confirmed by another study comparing cardiac differentiation of hESC-derived EBs on different ECM-derived matrices under hypoxia. Collagen IV and gelatin increased the overall number of beating EBs and enhanced the cardiac troponin T (cTNT) expression compared to fibronectin. These results revealed that collagen IV could potentially serve as a substrate for cardiogenesis [42]. In addition to collagen IV used in cardiogenesis ex vivo, the interaction between type I collagen and β1 integrin has been suggested to be critical for the growth and cardiac differentiation of mouse iPSC-derived EBs, indicating that collagen I may be a potential substrate for cardiogenesis as well [43]. Human BM-MSCs can be directed to the epidermal lineage on electrospun collagen/poly(L-lactic acid)-co-PCL (collagan/poly(L-lactic acid) (PLLA)/PCL) nanofibrous scaffolds by adding epidermis-inducing factors such as epidermal growth factor (EGF) and 1,25-dihydroxyvitamin D3. The coating of collagen on scaffolds significantly increased the number of cells grown on this nanofibrous scaffold. BM-MSCs differentiated on collagen/PLLA/PCL nanofibrous scaffold showed a round keratinocyte morphology and expressed keratin 10, filaggrin, and involucrin protein, indicating the cell commitment to epidermal lineage [44]. Human BM-MSCs were grown on tissue culture plate coated with type I collagen gel. Depth-dependent differentiation of hMSCs to the epithelial lineage was observed with the thick collagen gel (1900 μm), which generated significantly more cytokeratin 18 (CK18)-positive cells than collagen gel of a less thickness (100 μm). The addition of all-trans retinoic acid (ATRA) enhanced CK18 expression and induced cluster formation in cells grown on the thick collagen gel. The authors argued the effect of gel depth on hMSC differentiation was caused by partial cytoskeletal disruption, suggesting type I collagen gel can be combined with ATRA to differentiate hMSCs to epithelial cells [45]. Corneal epithelial-like cells were generated from hair follicle- or skin fibroblast-derived hiPSCs. When coupling with BMP4, collagen IV promoted the differentiation of iPSCs to epithelial cells with a high purity [46]. Collagen substrates also support stem-cell differentiation to hepatocyte-like cells and MSCs. Synergistically with hepatocyte growth factor (HGF), collagen I promoted the hepatocyte generation of human adipose-derived stem cells in 2 weeks, as evidenced by upregulation of α-fetoprotein, albumin, and α-antitrypsin [47]. A one-step method using collagen I was reported to obtain MSC-like cells from hESCs and iPSCs, which could provide a readily available multipotent cell source for tissue engineering. Dissociated human H9 ESCs and HDFa-YK26 iPSCs were cultured on thin, fibrillar collagen I coatings with ROCK inhibitor Y27632. Interestingly, collagen I reduced stem-cell attachment compared to the culture plastic. After incubation for 10 days, colonies of spindle-shaped cells with MSC marker expression were obtained and identified. This study demonstrates that type I collagen can be used to guide a rapid and efficient derivation of MSC-like cells from human pluripotent stem cells (hPSCs) [48]. As an important ECM protein, laminin has been reported to support the adhesion and functioning of a wide variety of stem cells [2, 49–52]. Of the different types of cell that can be differentiated on laminin, focus has been placed on neural cells. Laminin has been shown to promote NSC differentiation both in vitro and in vivo [50,51, 53–55]. The migration, expansion, and differentiation of human and mouse neural precursor cells (NPCs) into neurons and astrocytes were enhanced on laminin compared to fibronectin and Matrigel. The elongation of neurites from NPC-derived neurons was also observed on laminin. It was further revealed that the laminin-binding integrins α3, α6, α7, β1, and β4 expressed on these NPCs played a key role in laminin-dependent cell functions [51]. Again, the involvement of α6β1 integrin in laminin-induced neural progenitor expansion and neurite outgrowth was demonstrated [54, 56]. The direct incorporation of laminin in synthetic materials enhanced NSC attachment and functioning. Laminin-coated electrospun polyethersulfone fibers were shown to support the differentiation of rat NSCs to oligodendrocytes and neurons [57]. Laminin-1-coated methylcellulose scaffold promoted the survival and maturation of primary murine neurospheres with a significantly low level of apoptosis. The addition of laminin enhanced the expression of neuronal and oligodendrocyte precursor markers [56]. Poly-D-lysine (PDL) can be incorporated in laminin to provide a positively charged substrate and facilitate the differentiation of NSCs. For example, adult rat BM-MSCs were expanded on PDL- and laminin-1-coated glass in vitro, leading to the expression of nestin, a marker associated with neuronal progenitor cells, as compared to cells on TCPS- and PDL-coated surfaces, indicating laminin is crucial to NSC differentiation [58]. Similarly, PDL-laminin and Matrigel were reported to significantly promote the differentiation of hESC-derived EBs into neural progenitors, as well as to enhance neurite outgrowth compared to collagen, fibronectin, and PDL alone. Laminin was also found to stimulate the expansion and neurite outgrowth of hESC-derived neural progenitor cells in a dose-dependent manner [54]. Laminin-derived peptides can be conjugated to synthetic substrates and direct stem-cell differentiation. A laminin-derived IKVAV motif was conjugated to a self-assembling peptide, RADA16, to make a peptide-based scaffold. The extended IKVAV sequence directed the adhesion and neural differentiation of NSCs encapsulated in the scaffold. Histological analysis following injection in rat brain revealed the RADA-IKVAV peptide enhanced survival of encapsulated NSCs and reduced the formation of glial astrocytes [50]. In another study, the IKVAV motif was conjugated to poly(2-hydroxyethyl methacrylate) (PHEMA) with Ac-CGGASIKVAVS-OH peptide. Porous Ac-CGGASIKVAVS-OH-modified PHEMA scaffolds significantly promoted the cell attachment, proliferation, and differentiation of human fetal NSCs [53]. However, modifying an interpenetrating polymer network (IPN) with laminin-derived IKVAV peptide (CSRARKQAASIKVAVSADR) failed to support the adhesion and differentiation of adult NSCs, suggesting other factors such as substrate stiffness and ligand density might jointly influence the overall performance of laminin-derived peptide in regulating NSC function [59]. Laminin-coated surfaces are able to support cardiogenesis and osteogenesis. Compared to collagen IV-coated scaffolds, laminin-coated 3D scaffolds promoted the development of Flk+ cardiac progenitor cells from murine ESCs [41]. The late differentiation of cardiomyocytes from adipose-derived stem cells was enhanced on laminin, as demonstrated by a significant increase of sarcoendoplasmic reticulum calcium transport ATPase 2α (SERCA-2α) and myosin light chain 2α (MLC-2α) expression, as well as the number of desmin-positive cells after 5 weeks [60]. Laminin-modified poly(ethylene glycol) diacrylate (PEGDA) hydrogels increased the expression of osteogenic markers that might occur via the activation of α2, αv, and α6 integrin subunits [49]. Moreover, laminin-induced osteogenesis was reported to be mediated by focal adhesion kinase (FAK) and extracellular signal-regulated kinases 1 and 2 (ERK1/2) pathways triggered by the interaction between integrins and laminin [61].
Biomaterials for Directed Differentiation
11.1 Introduction
11.2 Natural Biomaterials
11.2.1 ECM-Derived Materials
11.2.1.1 Matrigel
11.2.1.2 Fibrin
11.2.1.3 Collagen
11.2.1.4 Laminin
11.2.2 Non-ECM-Derived Materials
11.2.2.1 Chitosan
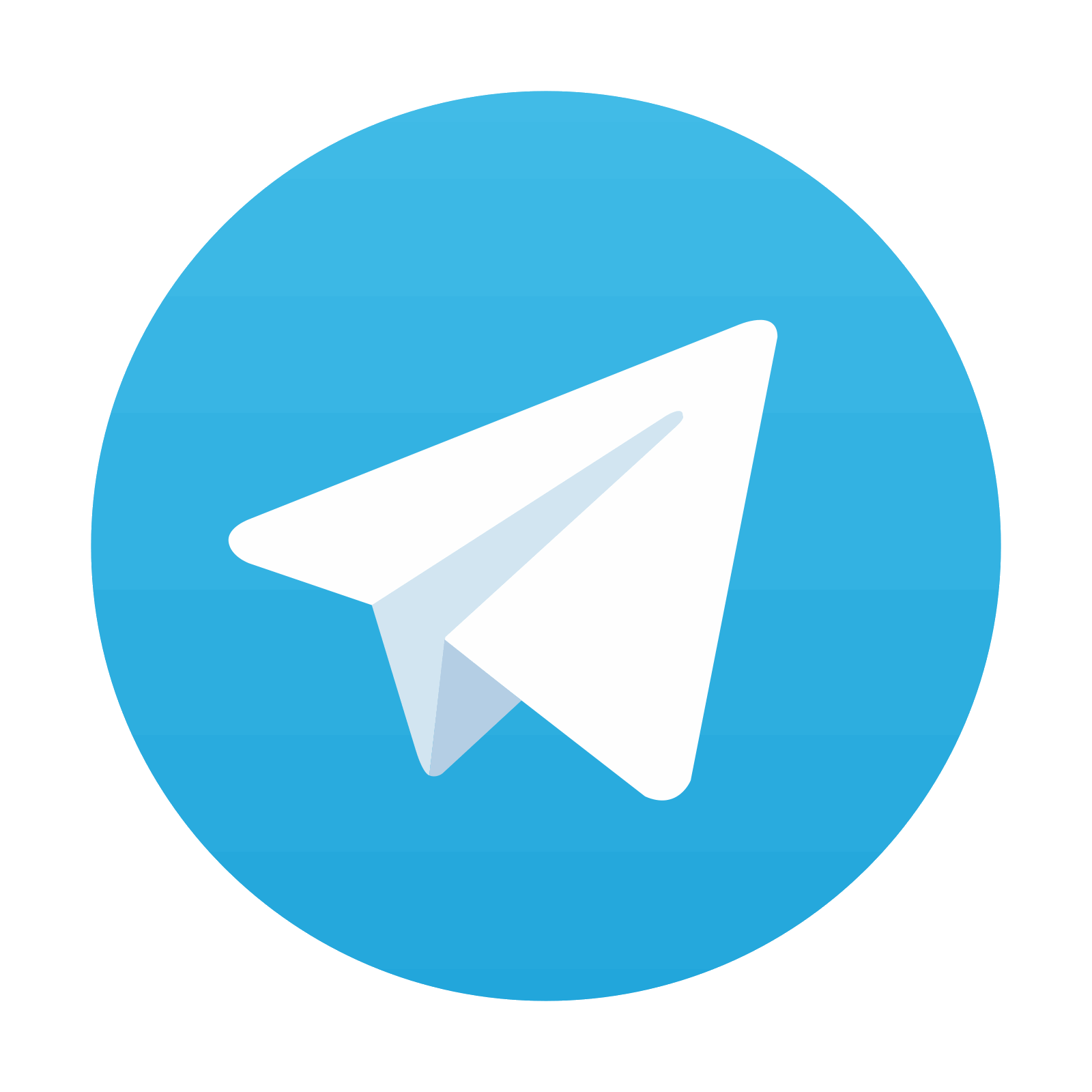
Stay updated, free articles. Join our Telegram channel
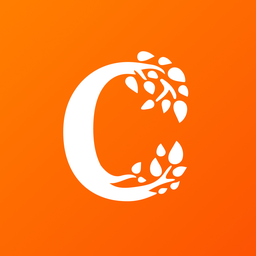
Full access? Get Clinical Tree
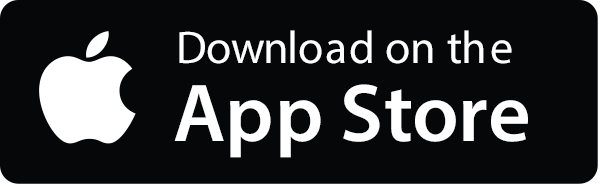
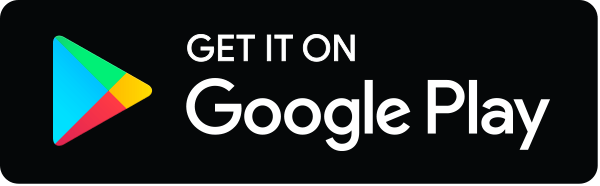