Biofilm and Biofilm Control
Joey S. Lockhart
Andre G. Buret
Douglas W. Morck
Microbial biofilms are ubiquitous, naturally occurring aggregates of microorganisms that are among the most successful life forms in the natural world. These unique communities can be composed of bacteria, fungi, and viruses and pose serious medical problems, particularly in the context of chronic infections and especially where medical implants are involved. Biofilms can occur in almost any aqueous or dry1,2 milieus and therefore are significant, persistent contaminates in most environments. The microorganisms in a biofilm are able to attach to almost any substrate. Examples of this include a rock in a stream, the hull of a ship, suture material or implanted medical device in a host, and the natural growth of intestinal bacteria. Biofilm communities are typically encased in polysaccharide capsules that contain various antigens, components of dead microorganisms (proteins, lipids, nucleic acids), inorganic and organic materials, and host-derived materials such as immune cells and DNA.3 Observations of biofilm-like structures date back to the 1670s with Antonie van Leeuwenhoek and his observations of dental plaque biofilms; however, the significance of the biofilm mode of growth was not fully appreciated until 1978, when J. William (Bill) Costerton and colleagues4 published their theories about this sessile form of bacterial growth. The observation that bacteria can and do exist as a community attached to a substrate, encased in a polysaccharide-rich matrix (referred to as glycocalyx by Costerton), was a critical step in the progression of biofilm research. Costerton noted that this was the predominant mode of growth in the vast majority of natural aquatic environments and concluded that these attached communities exhibit significant differences in physiology when compared to their planktonic (or free floating) counterparts.4 He suggested a phenotypic plasticity in biofilms5 with mechanisms that enable the bacteria to respond to environmental conditions and improve survival.6,7
Prior to the pioneering biofilm research by Costerton, in vitro work performed on bacteria considered the planktonic growth of microorganisms in liquid culture as the “gold standard” for studying bacterial physiology7 as well as virtually all medical and industrial applications. This is particularly true for the vast majority of research studying the bactericidal activity of various disinfectants, biocides, and antibiotics. Experiments investigating planktonic organisms do not necessarily provide valuable insight into the in situ efficacy of antimicrobial agents; and now, it is clear that in the natural world, microbes are more likely to exist as complex biofilm communities attached to various substrates in the environment.
Following these initial studies, scientists began exploring this distinctly different form of growth. Initial experiments investigating bacterial susceptibility to various antimicrobials immediately demonstrated that biofilm-associated bacteria are significantly more resilient to these treatments. The biofilm mode of growth involves a complex, multispecies microbial community, complete with water channels, which permit the delivery of nutrients throughout the biofilm. These channels help transport and allow bacteria to trap free nutrients for metabolic use. This also aids in the dissemination of communication molecules among species and between individual microbes within the biofilm community.

The physical characteristics of a biofilm allow for the development of antibiotic resistance through horizontal gene transfer events and inhibit the diffusion of various biocides into the center of the mature biofilms. This makes biofilms significantly more difficult to eradicate than planktonic organisms, which float freely within culture medium and have been most commonly studied in laboratory settings over the past century. In addition to presenting a physical barrier to disinfectants, microbes in a biofilm exhibit dramatic differences in gene expression
compared to their planktonic counterparts8 resulting in distinct phenotypes.
compared to their planktonic counterparts8 resulting in distinct phenotypes.
Biofilms form when planktonic bacteria are able to attach to a surface.9 Attached bacteria then produce an extracellular matrix typically consisting of polysaccharides, proteins, and DNA,10 which enables the bacteria to adhere irreversibly to the surface (Figure 67.1).9,11,12 These extracellular polysaccharides (EPS) are extensively hydrated, can impede diffusion of antimicrobials, and act as a physical barrier to host innate immune cells allowing the bacteria to cause persistent infections.9,13,14 The physiological generation of EPS is a critical feature of a biofilm and confers numerous advantages to the microorganisms residing in the biofilm community (Figure 67.2). One major benefit that the EPS layer provides to the community is resistance to desiccation because water stress is an environmental pressure that organisms frequently experience. The active production of EPS molecules makes biofilm bacteria significantly more resilient to desiccation than their planktonic counterparts (see Figure 67.2).16
![]() FIGURE 67.1 Biofilm life cycle. Free-floating planktonic bacteria adhere to various abiotic or biotic surfaces. At a certain cell density, bacteria exhibit changes in gene expression and will begin to produce signal molecules such as quorum sensing molecules. This results in the production of a thick extracellular polysaccharide layer around the microbial community, providing protection from host immune cells, desiccation, and an environment conducive to horizontal gene transfer. Planktonic bacteria are periodically released from the community to colonize new locations. Host immune cells such as neutrophils and macrophages are often unable to clear the microbial community and contribute to the structure of the biofilm. Adapted from Harrison et al11 and Stoodley et al.12 |
Diffusion of reactive oxidants such as hydrogen peroxide produced by host immune cells, or similar molecules used in disinfection treatments, can be limited and slowed such that the oxidants are inactivated prior to penetrating the outer layer of the biofilm.17,18 Antimicrobials administered for the treatment of infections can also be
inactivated before they effectively penetrate a biofilm.19 This diffusion barrier can create an environment that promotes the development of random mutations with the potential of conferring greater antimicrobial resistance to some of the bacteria. Constant low-level exposure to antimicrobial agents in particular can promote increased resistance development within a biofilm. In addition, the physical characteristics and three-dimensional structure of biofilms prevent diffusion of various biocides, and this mode of growth is typically more resistant to biocides than planktonic cells.14,20 The length of time that biofilms are permitted to grow and generate a more complex EPS enhances this resistance, as mature biofilms show increased tolerance to biocides such as glutaraldehyde.21 Biofilm-associated microbes experience environmental heterogeneity, meaning that different microniches within the biofilm have different concentrations of nutrients.15,22 At the center of a biofilm, the oxygen and other nutrient concentrations can be 30 times less than the concentrations at the surface.23,24 The conditions at the center of the biofilms can even be completely anaerobic in an otherwise aerobic environment.24 This change in nutrient availability appears to cause cells near the center of the biofilm to exist in a slow-growing state.9,25 Bacteria growing in this nutrient-restricted state are much less susceptible to antimicrobial agents because the mechanisms of many antimicrobials target the synthesis of nucleic acids and proteins in growing and dividing cells and minimally affect dormant cells that are not actively and rapidly producing these molecules.26 The differences in metabolic states of the cells within a biofilm ensure that some bacteria will survive exposure to antimicrobials9 or other harsh environmental challenging conditions. Channels for water and nutrients are present throughout the entire structure of the biofilm, which help the microbes near the center receive necessary nutrients. Certain populations of planktonic bacteria, known as “swimmers,” can tunnel into the biofilm, creating another distinct system of pores that help supply nutrients and oxygen to nutrientdeficient areas.24,27
inactivated before they effectively penetrate a biofilm.19 This diffusion barrier can create an environment that promotes the development of random mutations with the potential of conferring greater antimicrobial resistance to some of the bacteria. Constant low-level exposure to antimicrobial agents in particular can promote increased resistance development within a biofilm. In addition, the physical characteristics and three-dimensional structure of biofilms prevent diffusion of various biocides, and this mode of growth is typically more resistant to biocides than planktonic cells.14,20 The length of time that biofilms are permitted to grow and generate a more complex EPS enhances this resistance, as mature biofilms show increased tolerance to biocides such as glutaraldehyde.21 Biofilm-associated microbes experience environmental heterogeneity, meaning that different microniches within the biofilm have different concentrations of nutrients.15,22 At the center of a biofilm, the oxygen and other nutrient concentrations can be 30 times less than the concentrations at the surface.23,24 The conditions at the center of the biofilms can even be completely anaerobic in an otherwise aerobic environment.24 This change in nutrient availability appears to cause cells near the center of the biofilm to exist in a slow-growing state.9,25 Bacteria growing in this nutrient-restricted state are much less susceptible to antimicrobial agents because the mechanisms of many antimicrobials target the synthesis of nucleic acids and proteins in growing and dividing cells and minimally affect dormant cells that are not actively and rapidly producing these molecules.26 The differences in metabolic states of the cells within a biofilm ensure that some bacteria will survive exposure to antimicrobials9 or other harsh environmental challenging conditions. Channels for water and nutrients are present throughout the entire structure of the biofilm, which help the microbes near the center receive necessary nutrients. Certain populations of planktonic bacteria, known as “swimmers,” can tunnel into the biofilm, creating another distinct system of pores that help supply nutrients and oxygen to nutrientdeficient areas.24,27
![]() FIGURE 67.2 Properties of mature biofilms. The biofilm mode of growth provides several advantages to the microorganisms living in the community. The production of a hydrated extracellular matrix allows for increased nutrient acquisition and generates localized gradients that result in dramatic differences in nutrient exposure depending on where the organisms are located within the biofilm. This results in differences in growth rates of the microorganisms based on location and leads to the development of bacterial “persister” cells. A similar phenomenon is observed with the diffusion of antimicrobial molecules, and this helps promote the emergence of tolerance and resistance. The close proximity of bacteria to one another also aids in social interactions such as metabolite sharing between different species. Together, these properties make for a much more robust and resilient form of bacterial growth compared with traditional planktonic organisms. Reprinted by permission from Nature: Flemming et al.15 Copyright © 2016 Springer Nature. |
The frequency of mutation in biofilms is significantly higher than in planktonic organisms, leading to the development of antibiotic resistance and other genetic changes more rapidly in biofilms than in planktonic cells.13 Biofilmassociated bacteria exhibit what is termed a “hypermutable phenotype,” creating mutations in the DNA repair pathways leading to the enhanced expression of numerous mutant phenotypes within the community.13 This seems to be an evolutionary strategy for microbes to survive a variety of unique challenges by rapidly creating a diversity in their genotypes and phenotypes. Biofilm-associated bacteria are more resistant to various environmental stresses such
as desiccation, nutrient limitation, toxin actions, oxidative stresses, and heat shock through these mutations, and this hypermutable state can lead to increased antimicrobial resistance opportunities.13,26,28,29,30,31
as desiccation, nutrient limitation, toxin actions, oxidative stresses, and heat shock through these mutations, and this hypermutable state can lead to increased antimicrobial resistance opportunities.13,26,28,29,30,31
All biofilms found in the natural environment are polymicrobial (ie, composed of multiple microbial genera and species) because this provides numerous advantages to the microbes.32 Multiple species living together exhibit an increased rate of horizontal gene transfer (movement of genes from one bacterium to another), and this, in conjunction with the hypermutable phenotype, allows bacteria living within the biofilm to more efficiently deal with environmental stresses than any single species in planktonic form.33 Certain types of bacteria are able to promote the growth of other bacterial species when in proximity, such as in coculture. For example, the presence of Fusobacterium nucleatum in a culture allows Porphyromonas gingivalis to grow under microaerophilic conditions that would otherwise be toxic to monocultures of P gingivalis.34 The process of symbiotic nitrification is another example of metabolite cooperation in biofilms. In this phenomenon, specific bacteria from the genus Nitrospira oxidize ammonium to nitrite, which then is oxidized by other members of this genus to nitrate.35 These observations provide clear evidence for the reasons why bacteria aggregate and form polymicrobial communities that exhibit social interactions such as competition, cooperation, and synergism.
Just like in higher organisms, communication among individual cells is a critical aspect of microbial life in a complex community. All the processes described earlier rely on the exchange of information between microorganisms within a biofilm. Bacteria in biofilms communicate with one another via various signaling molecules in a process initially termed quorum sensing. Waters and Bassler36 comprehensively review the quorum sensing concept. Disruption of quorum sensing pathways may represent a powerful alternative to traditional antimicrobial treatments. For example, a recent study on aquatic, biofilm-forming species of Vibrio demonstrated that intermediates of polyhydroxy butyrates effectively inhibit biofilm formation in a strain-specific fashion by disrupting communication between cells.37 Such unique strategies may lead to novel mechanisms of inhibiting and killing microbes.
Biofilms are a significant issue in any system that relies on the movement of water or other nutrient-laden liquids through a pipeline, tube, or planar structure. The shear forces in these systems create environments that are conducive toward biofilm development and growth.31 Biofilms and their associated structures can act as a reservoir for pathogens in various medical devices that require water or other liquids, such as washer-disinfectors, and may result in relatively mild consequences such as water discoloration or, in catastrophic cases, can result in large outbreaks of infectious disease.38,39,40 Biofilms present a substantial problem in the water reservoirs and associated liquid delivery systems in surgical and medical equipment such as those used in eye surgery facilities.38 One particularly notable pathogen known to be capable of biofilm formation in air conditioner and domestic water supply cooling towers is Legionella pneumophila.40 This particular bacterium can cause serious outbreaks of pneumonia infections or a condition known as legionellosis or Legionnaires’ disease. Biofilms of Legionella within contaminated cooling towers are one of the earliest reported and major sources of infection in such outbreaks.40 These outbreaks are particularly severe in immunocompromised populations.41 New methods to detect bacteria growing in these systems include DNA amplification and next generation DNA sequencing, which have brought biofilms to the attention of researchers particularly in the field of water contamination.40,42
Up to 60% of clinical infections treated with antibiotics are estimated to have involved biofilms.8 Biofilm-associated infections can slowly lead to chronic tissue damage caused by the continual production or overproduction of proinflammatory molecules by frustrated host immune cells such as neutrophils (see Figure 67.1). Inflammationinduced tissue damage occurs in this fashion in conditions such as cystic fibrosis,43 periodontal disease,44 and a number of other chronic infectious diseases. In vivo experiments have shown neutrophil migration within biofilms. In some cases, the EPS matrix incorporates damaged and/or dead host immune cells, likely strengthening the barrier to other host immune cells (Figure 67.3).3 Quiescent biofilms act as nidi of future infection in a host; however, certain toxins (such as endotoxin; see chapter 66) and other bacterial products can be released periodically from mature biofilms that can result in immune responses and inflammation at near and distant locations. Biofilms growing in an environment such as the water reservoirs of medical washing-disinfection equipment can cause postsurgical aseptic inflammation in patients through the release of heat-resistant endotoxins. Such aseptic endotoxin-mediated inflammation events have been implicated in cases of diffuse lamellar keratitis (DLK) in patients undergoing corneal LASIK surgery38 as well as in outbreaks of toxic anterior segment syndrome (TASS).45 A combination of mechanical abrasion and biocide application was required to dramatically reduce the incidence of DLK38 and TASS caused by these biofilmcolonized surfaces.45

Biofilms form on a wide variety of medical devices, often resulting in chronic infections that are difficult to treat. Infections surrounding medical implants are a serious therapeutic challenge. The economic effect of such biofilm-associated infections is staggering, with estimates of up to 40 billion US dollars annually in the United States alone.46 The impact of biofilms in implant infections is reviewed by Costerton et al in 2005.47
![]() FIGURE 67.3 A, Light photomicrograph of an in vivo-generated biofilm, removed and stained with methylene blue and basic fuchsin and imaged 4 or 28 d after insertion into the peritoneal cavity of a rabbit. The biofilms on the implants were composed of both microbial proteins and host vascularized connective tissue. B, Transmission electron micrograph showing that live and degenerating host immune cells make up a significant proportion of the in vivo biofilm as early as 4 d after biofilm development. Visible in this image are host neutrophils, macrophages, erythrocytes, and a network of fibrin. From Buret et al.3 Copyright © 1991 John Wiley & Sons, Inc. Reprinted by permission of John Wiley & Sons, Inc. |
Biofilms are associated with infections on implanted artificial heart valves, orthopedic joint prostheses, catheters, stents, dental implants, contact lenses, and numerous other artificial substrates placed in or adjacent to host tissue.48,49,50,51,52 These implant-associated biofilms often result in chronic infections that require multiple treatments and, in severe cases, result in the removal of the afflicted implant.9,50,53
Suture material is at high risk of biofilm development from skin-based microorganisms, and this is a significant cause of surgical site infection (SSI)53 and surgical wound dehiscence. These types of infections are a heavy burden on the medical industry and can account for substantial proportions of the total hospital budget.54 In a large portion of these infections (up to 30%), clinicians are unable to culture any viable species from the wounds and this may be due to the biofilm mode of growth itself55 or the involvement of microorganisms that are challenging to culture such as mycobacteria.56 Similarly, SSIs may
involve bacteria that are slow to grow or are not typically scrutinized by medical diagnostic laboratories. Some researchers hypothesize that the slow-growing phenotype makes it more challenging to identify clinically relevant biofilm-associated bacteria because traditional clinical diagnostic approaches do not allow enough time for visible growth on culture plates.55 Biofilms composed of nontuberculous mycobacteria have also been implicated as causative agents of postsurgical infections, and these are very difficult to treat because many typical antibiotics or antimycobacterial drugs do not eradicate the bacteria.56
involve bacteria that are slow to grow or are not typically scrutinized by medical diagnostic laboratories. Some researchers hypothesize that the slow-growing phenotype makes it more challenging to identify clinically relevant biofilm-associated bacteria because traditional clinical diagnostic approaches do not allow enough time for visible growth on culture plates.55 Biofilms composed of nontuberculous mycobacteria have also been implicated as causative agents of postsurgical infections, and these are very difficult to treat because many typical antibiotics or antimycobacterial drugs do not eradicate the bacteria.56
Using confocal laser scanning microscopy (CLSM) and fluorescent in situ hybridization (FISH) on samples from 15 patients with SSIs associated with biofilm growth on permanent, multifilament sutures, investigators determined that the biofilms on the sutures were all polymicrobial in nature.53 These biofilms were composed of several microbial taxa, with Staphylococcus aureus (and in particular methicillin-resistant S aureus) being the most prevalent. An earlier case study demonstrated that a polymicrobial biofilm attached to sutures caused a persistent surgical wound infection in a patient.57 In these particular cases, which are likely to be more common than the literature suggests, treatments using antibiotics were not successful, and the sutures needed to be removed to eliminate the chronic infections.53,57 These studies illustrate several complicating issues with biofilm-associated chronic infections. Fortunately, removal of sutures is relatively simple. In other types of biofilm-associated infection, this is not a trivial task (eg, hip and knee joint prostheses or artificial heart valves). As described earlier, treatments with antimicrobials often fail due to lack of diffusion of the antimicrobial into biofilm, the increased incidence of gene transfer (that can convey resistance) within the biofilm as well as the presence of “persister” or metabolically dormant microbial cells in biofilms. The result is increased time spent in health care facilities, making patients even more susceptible to subsequent infections and most particularly when the individual is immunocompromised.

The generation of multiple, equivalent, and uniform biofilms for high-throughput in vitro studies is a significant challenge that microbiologists face. The major issue is reproducibility. There remains controversy among research groups regarding which method of biofilm generation in the laboratory provides the most accurate and realistic representation of biofilms and therefore several different techniques have become widely employed by groups around the globe. The use of a variety of methods creates challenges when reproducing previously published work. Therefore, standardization of experimental design, and perhaps even methods, is crucial in this important field of microbiological research. The following section briefly describes some of the common methods for biofilm generation in the laboratory, highlighting the advantages and disadvantages of each technique.
Static Systems
Peterson and colleagues58 review several different methods for the generation of biofilms. Initial experiments investigating biofilm growth relied on static approaches. This method was originally developed to measure adhesion of S aureus and has since been used to assess bacterial attachment and associated genes using mutants of several different bacterial species. Many studies have employed the static plate method to assess the effects of various natural and artificial inhibitors on overall biomass and biofilm formation.59,60,61 Although this approach is useful for quantifying the initial attachment of bacteria to an abiotic surface, the static method lacks the shear forces necessary for representative mature biofilm development. Additionally, this method does not allow users to supply fresh nutrients to the bacteria in the biofilm. With the limitations of these types of in vitro assays, many researchers advise caution when drawing conclusions and extrapolating results to in vivo and natural biofilms. Results from a study on Enterococcus faecalis biofilms (organisms that play a significant role in endocarditis) indicated that biofilms generated using a static method on polystyrene plates were not equivalent to biofilms grown on porcine heart valves.62 This led the researchers to conclude that E faecalis biofilms generated in vitro are not clinically relevant and suggested that these types of studies should be validated using ex vivo approaches prior to publication.62 Despite such limitations, the static microplate growth method does allow scientists to quantify the biomass of various biofilms and as such, this method is still widely accepted as a tool to assess biofilm growth.58,63,64,65 This quick method to assess the total biomass in the wells of microplates using crystal violet is colloquially referred to as the microtiter dish biofilm assay, or O’Toole assay.66
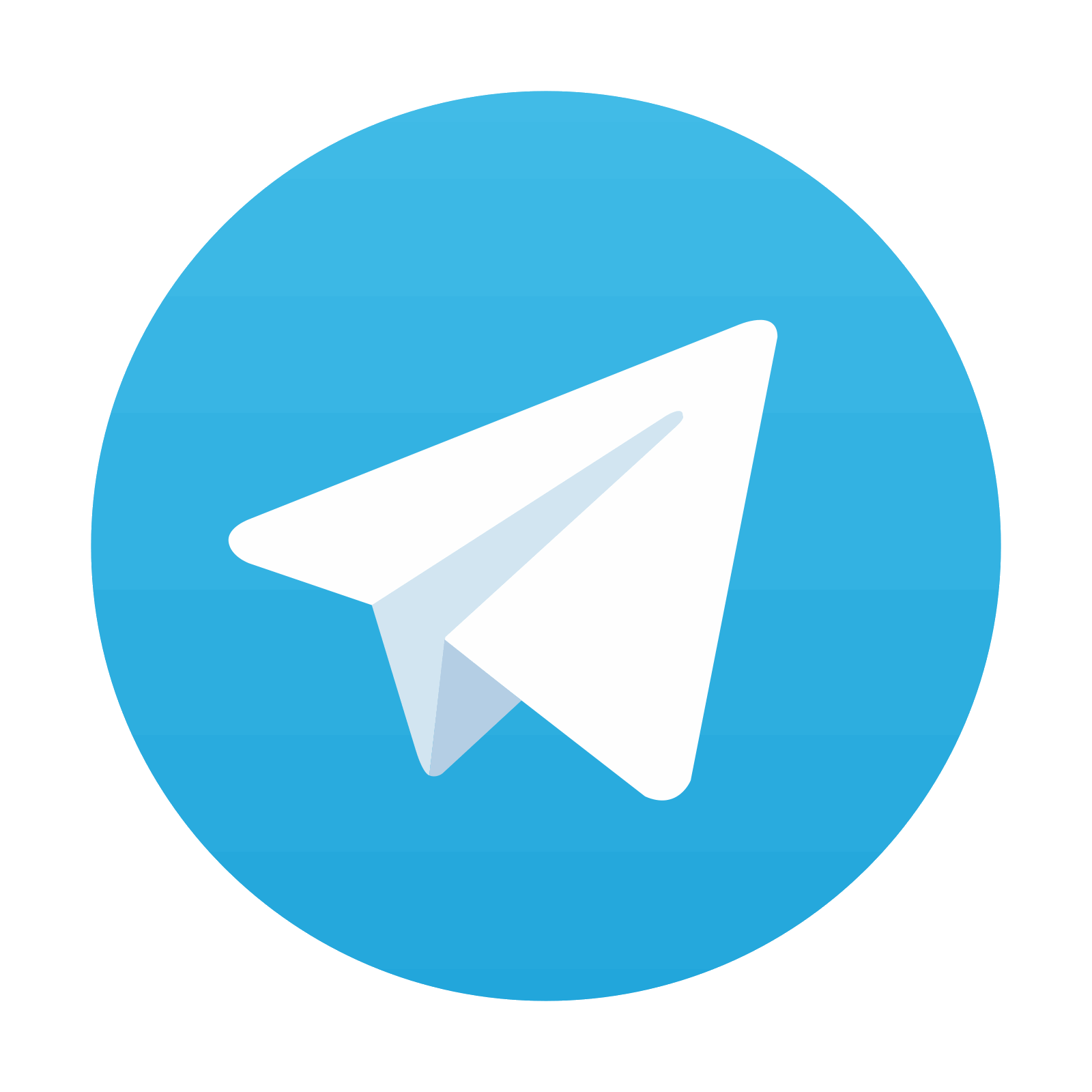
Stay updated, free articles. Join our Telegram channel
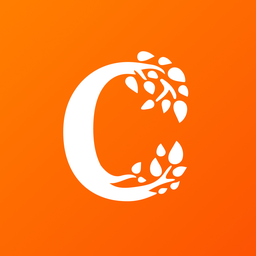
Full access? Get Clinical Tree
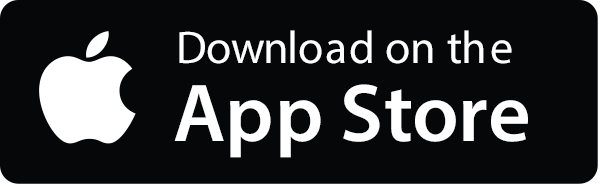
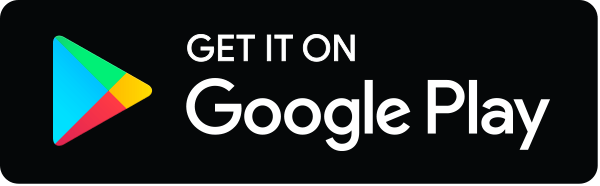