Each of us is composed of a large number of complex molecules that are hierarchically arranged in space to form cells, tissues, organs, and ultimately a complete human being. These molecules are constructed from individual elements that may be synthesized endogenously or obtained from the environment. Once created these molecules are not static. In fact they are perpetually being synthesized, degraded, excreted, and sometimes recycled in a tightly choreographed metabolic dance.
Each metabolic process consists of a sequence of catalytic steps mediated by enzymes encoded by genes. Usually these genes are replicated with high fidelity, and enzymatic systems continue to work effectively from generation to generation. Occasionally, mutations reduce the efficiency of encoded enzymes to a level at which normal metabolism cannot occur. Such variants of metabolism were recognized by Sir Archibald Garrod at the beginning of the 20th century, based partly on his studies of alkaptonuria (AKU). Garrod recognized that these variants illustrated “chemical individualities,” and he called these disorders “inborn errors of metabolism,” thus setting the cornerstone for contemporary biochemical genetics.
AKU is a rare disorder in which homogentisic acid (HGA), an intermediate metabolite in phenylalanine and tyrosine metabolism ( Fig. 7.1 ), is excreted in large quantities in urine, causing it to darken on standing. Hence AKU was classically referred to as “black urine disease.” Additionally, an oxidation product of HGA is directly deposited in connective tissues, resulting in abnormal pigmentation and debilitating arthritis.

Garrod proposed in 1902 that AKU was caused by a deficiency of the enzyme that normally splits the aromatic ring of HGA. Fifty years later it was established that AKU is produced by a failure to synthesize homogentisate 1,2-dioxygenase (HGO). However, it was not until 1996 that scientists identified the gene that is altered in AKU, based on homology to a gene encoding an HGO enzyme that was isolated from a fungus species. The coding region of HGO comprises 14 exons distributed over 54 kb of DNA. Many of the mutations identified in HGO encode proteins that show no HGO activity when expressed in vitro. This indicates that AKU is caused by loss-of-function mutations, confirming the hypothesis put forth by Garrod more than a century ago.
Almost all biochemical processes of human metabolism are catalyzed by enzymes. Variations of enzymatic activity among humans are common, and a minority of these variants causes disease. These concepts were introduced by Archibald Garrod and exemplified by his studies of alkaptonuria.
Variants of Metabolism
Prevalence of Metabolic Disease
Hundreds of different inborn errors of metabolism have been described to date, and most of these are rare. Taken together, however, metabolic disorders account for a substantial percentage of the morbidity and mortality directly attributable to genetic disease ( Table 7.1 ). One survey conservatively estimated that the incidence of metabolic disorders is approximately 1 in every 2500 births, or 10% of all monogenic conditions in children. Moreover, we are beginning to understand that different alleles of genes that encode enzymes can alter one’s risk for many common diseases, such as diabetes, heart disease, stroke, and cancer.
Name | Prevalence | Mutant Gene Product | Chromosomal Location |
---|---|---|---|
Carbohydrate Disorders | |||
Classic galactosemia | 1/35,000 to 1/60,000 | Galactose-1-phosphate uridyl transferase | 9p13 |
Hereditary fructose intolerance | 1/20,000 | Fructose 1,6-bisphosphate aldolase | 9q13-q32 |
Fructosuria | ≈1/100,000 | Fructokinase | 2p23 |
Hypolactasia (adult) | Common | Lactase | 2q21 |
Diabetes mellitus type 1 | 1/400 (Europeans) | Multiple | Polygenic |
Diabetes mellitus type 2 | 1/20 | Multiple | Polygenic |
Maturity-onset diabetes of the young (MODY) | ≈1/400 | Multiple | Multiple loci |
Amino Acid Disorders | |||
Phenylketonuria | 1/10,000 | Phenylalanine hydroxylase | 12q24 |
Tyrosinemia (type 1) | 1/100,000 | Fumarylacetoacetate hydrolase | 15q23-25 |
Maple syrup urine disease | 1/180,000 | Branched-chain α-ketoacid dehydrogenase (multiple subunits) | Multiple loci |
Alkaptonuria | 1/250,000 | Homogentisic acid oxidase | 3q2 |
Homocystinuria | 1/340,000 | Cystathionine β-synthase | 21q2 |
Oculocutaneous albinism | 1/35,000 | Tyrosinase | 11q |
Cystinosis | 1/100,000 | CTNS | 17p13 |
Cystinuria | 1/7000 | SLC3A1 (type 1) | 2p |
SLC7A9 (types II & III) | 19q13 | ||
Lipid Disorders | |||
MCAD | 1/1000 to 1/15,000 | Medium-chain acyl-CoA dehydrogenase | 1p31 |
VLCAD | 1/30,000 | Very Long-chain acyl-CoA dehydrogenase | 17p13.1 |
SLO | 1/10,000 | Δ7-sterol reductase | 11q12-q13 |
Organic Acid Disorders | |||
Methylmalonic acidemia | 1/20,000 | Methylmalonyl-CoA mutase | 6p |
Propionic acidemia | Rare | Propionyl-CoA carboxylase | 13q32; 3q |
Urea Cycle Defects | |||
Ornithine transcarbamylase deficiency | 1/60,000 | Ornithine carbamyl transferase | Xp21 |
Carbamyl phosphate synthetase deficiency | 1/300,000 | Carbamyl phosphate synthetase I | 2p |
Argininosuccinic acid synthetase deficiency | 1/250,000 | Argininosuccinic acid synthetase | 9q34 |
Energy Production Defects | |||
Cytochrome c oxidase deficiency | Rare | Cytochrome oxidase peptides | Multiple loci |
Pyruvate carboxylase deficiency | Rare | Pyruvate carboxylase | 11q |
Pyruvate dehydrogenase complex (E 1 ) deficiency | Rare | Pyruvate decarboxylase, E 1 α | Xp22 |
NADH-CoQ reductase deficiency | Rare | Multiple nuclear genes | Multiple loci |
Heavy Metal Transport Defects | |||
Wilson disease | 1/50,000 | ATP7B | 13q14 |
Menkes disease | 1/250,000 | ATP7A | Xq13 |
Hemochromatosis | 1/200 to 1/400 (Europeans) | HFE | 6p21 |
Acrodermatitis enteropathica | Rare | SLC39A4 | 8q24 |
The diagnosis of a metabolic disorder can be challenging ( Clinical Commentary 7.1 ); thus the morbidity associated with metabolic defects is probably underestimated. In the 1970s an often fatal acute metabolic encephalopathy and hepatopathy called Reye syndrome was diagnosed in many children. In the following decades, we learned that some children with an encephalopathy indistinguishable from Reye syndrome had a urea cycle defect that produced hyperammonemia (increased levels of circulating ammonia) and death. Recognition of Reye syndrome as a phenocopy of a urea cycle defect is important, because in addition to supportive care, ∗ the children can now receive direct treatment for the urea cycle defects. Similarly, on postmortem examination, some children who have died from sudden infant death syndrome (SIDS) have been found to have a defect of fatty acid metabolism. These are also treatable disorders, and life-threatening episodes can be avoided with appropriate care.
∗ Supportive care is care that supports the elementary functions of the body, such as maintenance of fluid balance, oxygenation, and blood pressure, but is not aimed at treating the disease process directly.
Although individual metabolic disorders are rare, their overall direct and indirect contribution to morbidity and mortality is substantial.
The presentations of persons with inborn errors of metabolism are highly variable. During gestation, the maternal–placental unit usually provides essential nutrients and prevents the accumulation of toxic substrates. Thus a fetus is infrequently symptomatic. However, after birth persons with metabolic disorders can present at ages ranging from the first day of life to adulthood. The presentation may be precipitous and characterized by dramatic alterations in homeostasis and even death. In contrast, the disorder may be insidious, with only subtle changes in function over long periods. For most metabolic disorders, the presymptomatic period and onset of symptoms lie somewhere between these two extremes. The following case illustrates this point.
Anthony is a 9-month-old Latin American boy who comes to the emergency department accompanied by his parents. His parents complain that he has been irritable and vomiting for the last 36 hours, and over the past 12 hours he has become increasingly sleepy. They sought medical attention because it was difficult to awaken Anthony to breast-feed him. Anthony’s medical history is unremarkable. He has a healthy 8-year-old sister and had a brother who died in his crib at 7 months of age. An investigation of the brother’s death and an autopsy were performed. The findings were consistent with sudden infant death syndrome (SIDS).
Anthony is hospitalized and is noted to be hypoglycemic (low plasma glucose level), slightly acidemic (plasma pH < 7.4), and hyperammonemic (elevated plasma ammonia). Intravenous infusion of glucose transiently improves his level of alertness, but he becomes comatose and dies 5 days later. An autopsy reveals marked cerebral edema (swelling of the brain) and fatty infiltration of the liver consistent with a diagnosis of Reye syndrome. Anthony’s mother is concerned that the boys’ deaths are related to each other, especially since she is pregnant again. She is counseled that the causes of death are unrelated and neither disorder is likely to recur in her family.
One year later her 6-month-old daughter, Maria, is hospitalized for the third time because of lethargy and weakness. Laboratory studies reveal moderate hypoglycemia, hyperammonemia, and ketonuria (ketones in the urine). Additional studies, including measurement of urine organic acids, ∗
∗ Organic acids are carbon-based acids that are products of intermediate metabolism and normally do not accumulate in plasma or urine beyond the buffering capacities of these fluids.
plasma amino acids, and free and esterified plasma carnitines, suggest that Maria has a defect of fatty acid oxidation. Therapy is initiated with intravenous glucose, oral carnitine, and avoidance of fasting. Genetic testing confirms that Maria has medium-chain acyl-CoA dehydrogenase (MCAD) deficiency, and testing of preserved tissues collected at autopsy from Maria’s deceased brothers indicate that they also had MCAD deficiency. Maria’s asymptomatic older sister is similarly affected. Both girls are healthy 2 years later. They have a new baby brother who underwent prenatal genetic testing for MCAD and was found to be unaffected.The disparate presentations of MCAD deficiency in this family (sudden death, acute illness, chronic illness, and asymptomatic) illustrate the phenotypic variability often observed in persons with inborn errors of metabolism, even those sharing an identical mutation. Thus there might not be a disease-specific pattern of symptoms and findings. Often it is the heightened index of suspicion of care providers that leads to the testing necessary to identify a metabolic disorder. Supportive therapy can be lifesaving and should be initiated before making a diagnosis. Nevertheless, it is imperative that prudent attempts be made to make a specific diagnosis, because it can have important implications for the family (e.g., prenatal testing, presymptomatic therapy). The treatment of MCAD deficiency is very effective in preventing early death.
Inheritance of Metabolic Defects
Most metabolic disorders are inherited in an autosomal recessive pattern; only individuals having two mutant alleles are affected. Although a mutant allele produces reduced or no enzyme activity (loss of function), it usually does not alter the health of a heterozygous carrier. Because many of the genes encoding disease-related enzymes have been identified and their mutations characterized, carrier testing and prenatal diagnosis are available for many metabolic disorders. However, testing samples of dried blood for elevated levels of metabolites in the newborn period (e.g., for phenylketonuria and galactosemia; see Chapter 13 ) remains the most commonly used population-based screening test for metabolic disorders. Expanded newborn screening tests for dozens of different disorders by checking for the presence of abnormal metabolites in blood. As the technology for rapid and efficient DNA testing of mutant alleles progresses, population-based screening for additional disorders is likely to be incorporated.
Most inborn errors of metabolism are inherited in an autosomal recessive pattern. The carrier state usually is not associated with morbidity. Carrier and diagnostic testing are becoming widely available for many disorders.
Types of Metabolic Processes
Metabolic disorders have been classified in many different ways, based on the pathological effects of the pathway blocked (e.g., absence of end product, accumulation of substrate); different functional classes of proteins (e.g., receptors, hormones); associated cofactors (e.g., metals, vitamins); and pathways affected (e.g., glycolysis, citric acid cycle). Each of these has advantages and disadvantages, and none of them encompasses all metabolic disorders. However, the classification that most completely integrates our knowledge of cell biology, physiology, and pathology with metabolic disorders categorizes defects of metabolism by the types of processes that are disturbed.
Defects of Metabolic Processes
Almost all biochemical reactions in the human body are controlled by enzymes, which act as catalysts. The catalytic properties of enzymes typically increase reaction rates by more than a million-fold. These reactions mediate the synthesis, transfer, use, and degradation of biomolecules to build and maintain the internal structures of cells, tissues, and organs. Biomolecules can be categorized into four primary groups: nucleic acids, proteins, carbohydrates, and lipids. The major metabolic pathways that metabolize these molecules include glycolysis, citric acid cycle, pentose phosphate shunt, gluconeogenesis, glycogen and fatty acid synthesis and storage, degradative pathways, energy production, and transport systems. We now discuss how defects in each of these metabolic pathways can cause human disease.
Carbohydrate Metabolism
Because of the many different applications that they serve in all organisms, carbohydrates are the most abundant organic substance on Earth. Carbohydrates function as substrates for energy production and storage, as intermediates of metabolic pathways, and as the structural framework of DNA and RNA. Consequently, carbohydrates account for a major portion of the human diet and are metabolized into three principal monosaccharides: glucose, galactose, and fructose. Galactose and fructose are converted to glucose before glycolysis. The failure to effectively use these sugars accounts for the majority of the inborn errors of human carbohydrate metabolism.
Galactose
The most common monogenic disorder of carbohydrate metabolism, galactosemia (classic galactosemia), affects 1 in every 50,000 newborns. It is most commonly caused by mutations in the gene encoding galactose-1-phosphate uridyl transferase (GAL-1-P uridyl transferase) ( Fig. 7.2 ). This gene is composed of 11 exons distributed across 4 kb of DNA, and approximately 70% of galactosemia-causing alleles in people of Western European origin have a single missense mutation in exon 6, p.Q188R. As a result of diminished GAL-1-P uridyl transferase activity, affected persons cannot effectively convert galactose to glucose; consequently, galactose is alternatively metabolized to galactitol and galactonate (see Fig. 7.2 ). Although galactose and its metabolites accumulate in many tissues, the pathophysiology of classic galactosemia is not well understood.

Classic galactosemia typically manifests in the newborn period with poor sucking, failure to thrive, and jaundice. If galactosemia is left untreated, sepsis, hyperbilirubinemia, coagulopathy, hepatocellular damage, and shock leading to death usually follow. Cataracts (opacification of the lens of the eye) are found in about 30% of infants but often resolve with treatment. Newborn screening for galactosemia is widespread, and most affected persons are identified as they begin to develop symptoms. Early identification affords prompt treatment, which consists largely of eliminating dietary galactose. This substantially reduces the morbidity associated with the acute effects of elevated levels of galactose metabolites. Long-term disabilities may include poor growth, developmental delay, † speech problems, and primary ovarian failure in females. These sequelae are thought to be caused by endogenous production of galactose. The effects of prospective dietary therapy on the prevalence of these long-term sequelae are less clear. Early studies suggested that there was no effect, but as more longitudinal data become available, it appears that patients treated early in life with dietary therapy may have a normal cognitive outcome.
† Developmental delay is the delayed attainment of age-appropriate motor, speech, and/or cognitive milestones; the outcomes of developmental delay range from normal to profound intellectual disability.
Galactosemia can also be caused by mutations in the genes encoding galactokinase or uridine diphosphate galactose-4-epimerase (UDP-galactose-4-epimerase) (see Fig. 7.2 ). Deficiency of galactokinase is associated with the formation of cataracts but does not cause growth failure, intellectual disability, or hepatic disease. Dietary restriction of galactose is also the treatment for galactokinase deficiency. Deficiency of UDP-galactose-4-epimerase can be limited to red blood cells and leukocytes, causing no ill effects, or it can be systemic and produce symptoms similar to those of classic galactosemia. Treatment is aimed at reducing the dietary intake of galactose, but not as severely as in patients with classic galactosemia, because some galactose must be provided to produce UDP-galactose for the synthesis of some complex carbohydrates.
Galactosemia is one of the most common inherited disorders of carbohydrate metabolism. Newborn screening for galactosemia is widespread. Early identification allows prompt treatment, which consists largely of eliminating dietary galactose. Mutations in the gene that encodes GAL-1-P uridyl transferase are the most common cause of galactosemia.
Fructose
Three autosomal recessive defects of fructose metabolism have been described. The most common is caused by mutations in the gene encoding hepatic fructokinase. This enzyme catalyzes the first step in the metabolism of dietary fructose, the conversion to fructose-1-phosphate ( Fig. 7.3 ). Inactivation of hepatic fructokinase results in asymptomatic fructosuria (presence of fructose in the urine).

In contrast, hereditary fructose intolerance (HFI) results in poor feeding, failure to thrive, hepatic and renal insufficiency, and death. HFI is caused by a deficiency of fructose 1,6-bisphosphate aldolase in the liver, kidney cortex, and small intestine. Infants and adults with HFI are asymptomatic unless they ingest fructose or sucrose (a sugar composed of fructose and glucose). Infants who are breast-fed become symptomatic after weaning, when fruits and vegetables are added to their diet. Affected infants may survive into childhood because they avoid foods they consider noxious, thereby self-limiting their intake of fructose. The prevalence of HFI may be as high as 1 in 20,000 births, and since the cloning of the gene encoding fructose-1-phosphate aldolase, differences in the geographic distribution of mutant alleles have been found.
Deficiency of hepatic fructose 1,6-bisphosphatase (FBPase) causes impaired gluconeogenesis, hypoglycemia (reduced level of circulating glucose), and severe metabolic acidemia (serum pH <7.4). Affected infants commonly present for treatment shortly after birth, although cases diagnosed later in childhood have been reported. If patients are adequately supported beyond childhood, growth and development appear to be normal. A handful of mutations have been found in the gene encoding FBPase, some of which encode mutant proteins that are inactive in vitro.
Asymptomatic deficiency of fructokinase is the most common defect of fructose metabolism. Hereditary fructose intolerance is less prevalent but is associated with much more severe problems.
Glucose
Abnormalities of glucose metabolism are the most common errors of carbohydrate metabolism. However, the causes of these disorders are heterogeneous and include both environmental and genetic factors. Historically, disorders associated with elevated levels of plasma glucose (hyperglycemia) have been classified into three categories: type 1 diabetes mellitus (T1DM), type 2 diabetes mellitus (T2DM), and maturity-onset diabetes of the young (MODY), of which there are many subtypes. T1DM is associated with reduced or absent levels of plasma insulin and usually manifests in childhood. T2DM is characterized by insulin resistance, and most commonly, adult onset. A more detailed discussion of T1DM and T2DM can be found in Chapter 12 .
Substantial advances in understanding of the pathophysiology of common diabetes have been achieved by identifying mutations that cause rare monogenic forms of hyperglycemia. Mutations in the insulin receptor gene have been associated with a disorder characterized by insulin resistance and acanthosis nigricans (hypertrophic skin with a corrugated appearance). These mutations can decrease the number of insulin receptors on a cell’s surface, or they can decrease the insulin-binding activity level or the insulin-stimulated tyrosine kinase activity level. Mutations in mitochondrial DNA and in the genes encoding insulin and glucokinase have also been associated with hyperglycemic disorders.
Studies of rare, monogenic forms of diabetes define the pathways that may be disturbed in the more common forms of diabetes mellitus.
Lactose
The ability to metabolize lactose (a sugar composed of glucose and galactose) depends, in part, on the activity of an intestinal brush-border enzyme called lactase-phlorizin hydrolase (LPH). In most mammals, LPH activity diminishes after infants are weaned from maternal milk. However, the persistence of intestinal LPH activity is a common autosomal recessive trait in human populations, with an incidence ranging from 5% to 90%. The geographic distribution of lactase persistence is concordant with areas of high milk intake, such as northwestern Europe and certain parts of Africa. The persistent ability for adults to use dairy products as a source of vitamin D had a selective advantage in these populations.
Lactase nonpersistence (i.e., adult-type hypolactasia or lactose intolerance) is common in most tropical and subtropical countries. Persons with lactase nonpersistence can experience nausea, bloating, and diarrhea after ingesting lactose. Thus in many regions where reduced lactase activity is prevalent, the lactose in dairy products is often partially metabolized (e.g., by lactobacilli in the preparation of yogurt) before consumption. The role of lactase nonpersistence as a cause of abdominal pain and symptoms of irritable bowel syndrome is controversial.
LPH is encoded by the lactase gene (LCT) on chromosome 2. In European populations, adult LPH expression is regulated by a polymorphism located in an upstream gene named minichromosome maintenance 6 (MCM6). However, in sub-Saharan African, Central Asian, and Arabian peninsular populations in which lactase persistence is common, this polymorphism is found at low frequency or is absent. Lactase persistence in these populations is caused by different polymorphisms that appear to increase transcription of LCT. These polymorphisms appear to have arisen relatively recently in human evolution and have increased in incidence as a result of natural selection independently in populations in Europe and Africa. In each case, the selective force appears to have been a local adaptive response to the higher fitness afforded by the ability to consume dairy products.
Mutations that abolish lactase activity altogether cause congenital lactase deficiency and produce severe diarrhea and malnutrition in infancy. Such mutations are very rare.
Glycogen
Carbohydrates are most commonly stored as glycogen in humans. Consequently, enzyme deficiencies that lead to impaired synthesis or degradation of glycogen are also considered disorders of carbohydrate metabolism. Defects of each of the proteins involved in glycogen metabolism have been identified ( Table 7.2 ). These cause different forms of glycogen storage disorders and are classified numerically according to the chronological order in which their enzymatic basis was described. The two organs most severely affected by glycogen storage disorders are the liver and skeletal muscle. Glycogen storage disorders that affect the liver typically cause hepatomegaly (enlarged liver) and hypoglycemia (low plasma glucose level). Glycogen storage disorders that affect skeletal muscle cause exercise intolerance, progressive weakness, and cramping. Some glycogen storage disorders, such as Pompe disease, can also affect cardiac muscle, causing cardiomyopathy and early death. Treatment of some glycogen storage disorders by enzyme replacement (e.g., giving active forms of the enzyme intravenously) can improve, and in some cases prevent, symptoms and therefore preserve function and prevent early death.
Each disorder of glycogen metabolism is uncommon, yet taken together these defects account for substantial morbidity. Early intervention can prevent severe disabilities and early death.
Type | Defect | Major Affected Tissues |
---|---|---|
Ia (Von Gierke) | Glucose-6-phosphatase | Liver, kidney, intestine |
Ib | Microsomal glucose-6-phosphate transport | Liver, kidney, intestine, neutrophils |
II (Pompe) | Lysosomal acid β-glucosidase | Muscle, heart |
IIIa (Cori) | Glycogen debranching enzyme | Liver, muscle |
IIIb | Glycogen debranching enzyme | Liver |
IV (Anderson) | Branching enzyme | Liver, muscle |
V (McArdle) | Muscle phosphorylase | Muscle |
VI (Hers) | Liver phosphorylase | Liver |
VII (Tarui) | Muscle phosphofructokinase | Muscle |
Amino Acid Metabolism
Proteins play the most diverse roles of the major biomolecules (e.g., providing mechanical support, coordinating immune responses, generating motion). Indeed, nearly all known enzymes are proteins. The fundamental structural units of proteins are amino acids. Some amino acids can be synthesized endogenously (nonessential), and others must be obtained from the environment (essential). Many defects of the metabolism of amino acids have been described.
Phenylalanine
Defects in the metabolism of phenylalanine (an essential amino acid) cause the hyperphenylalaninemias, some of the most widely studied of all inborn errors of metabolism. These disorders are caused by mutations in genes that encode components of the phenylalanine hydroxylation pathway (see Fig. 7.1 ). Elevated levels of plasma phenylalanine disrupt essential cellular processes in the brain such as myelination and protein synthesis, eventually producing severe intellectual disability. Most cases of hyperphenylalaninemia are caused by mutations of the gene that encodes phenylalanine hydroxylase (PAH), resulting in classic phenylketonuria (PKU). Hundreds of different mutations have been identified in PAH, including substitutions, insertions, and deletions. The prevalence of hyperphenylalaninemia varies widely among groups from different geographic regions; PKU ranges from 1 in every 10,000 people of European origin to 1 in every 90,000 in those of African ancestry. Less commonly, hyperphenylalaninemia is caused by defects in the synthesis of tetrahydrobiopterin, a cofactor necessary for the hydroxylation of phenylalanine, or by a deficiency of dihydropteridine reductase.
Treatment of most hyperphenylalaninemias is aimed at restoring normal blood phenylalanine levels by restricting dietary intake of phenylalanine-containing foods (see Box 7.1 ). However, phenylalanine is an essential amino acid, and adequate supplies are necessary for normal growth and development. A complete lack of phenylalanine is fatal. Thus a fine balance must be maintained between providing enough protein and phenylalanine for normal growth and preventing the serum phenylalanine level from rising too high. Persons with PKU clearly benefit from life-long treatment. Thus once PKU is diagnosed, the person must follow a phenylalanine-restricted diet for life. Enzyme substitution therapy was recently approved for adults with PKU and is effective in helping reduce phenylalanine levels in patients in whom dietary restriction alone is not adequate. Sapropterin dihydrochloride, a form of tetrahydrobiopterin (BH4), can help BH-4 responsive PKU patients.
The most important component of therapy for many inborn errors of metabolism is manipulation of the diet. This commonly includes restriction of substrates that are toxic, such as carbohydrates (e.g., in galactosemia, diabetes mellitus), fats (e.g., in medium-chain acyl-coenzyme A dehydrogenase deficiency), and amino acids (e.g., in phenylketonuria [PKU], maple syrup urine disease, urea cycle defects); avoidance of fasting; replacement of deficient cofactors (e.g., B vitamins, carnitine); or using alternative pathways of catabolism to eliminate toxic substances. Because many metabolic disorders are diagnosed in infants whose nutritional requirements change quickly (sometimes weekly), it is imperative to provide infants with diets that provide adequate calories and nutrients for normal growth and development. Thus the responsibility for maintaining a special diet begins with the parents of an affected child and shifts to the child when he or she is capable of managing independently. For most persons with metabolic diseases, a special diet must be maintained for life. This results in many unique challenges that are often unforeseen by families and care providers alike. Consequently, it is important that support and guidance be provided by clinical dietitians, gastroenterologists, psychologists, genetic counselors, and biochemical geneticists.
For example, newborns with PKU are fed a low-phenylalanine diet to prevent the effects of hyperphenylalaninemia on the brain. Breast milk contains too much phenylalanine to be used as the only source of nutrients. Therefore many infants are fed an expensive low-phenylalanine formula that is available only by prescription, a medical food. ∗ Small quantities of breast milk can be mixed with the formula, although the breast milk must be pumped and carefully titrated to avoid giving the infant too much phenylalanine. Serum phenylalanine levels are measured frequently, and adjustments must be made to the diet to compensate for an infant’s growth and varying individual tolerances for phenylalanine. These interventions can disrupt mother–infant bonding and distort a family’s social dynamics.
As a child with PKU grows older, low-protein food substitutes are introduced to supplement the formula (e.g., low-protein breads and pasta). To put this in perspective, consider that a 10-year-old child with PKU might ingest 300 to 500 mg of phenylalanine per day. Thus three or four slices of regular bread would meet a child’s nutritional needs and dietary phenylalanine limit because of the relatively high protein content of grains. Low-protein foods make the diet more substantive and varied. Indeed, seven slices of low-protein bread contain the phenylalanine equivalent of one piece of regular bread. Yet, many of these foods have an odor, taste, texture, or appearance that differs distinctively from foods containing normal amounts of protein.
The intake of many fruits, fats, and carbohydrates is less restricted (see table at the right). However, phenylalanine is found unexpectedly in many food items (e.g., gelatin, beer). In fact, the FDA requires manufacturers to label products containing aspartame (a common artificial sweetener that contains phenylalanine) with a warning for persons with PKU.
Teenagers with PKU sometimes have difficulty socializing with their peers because their dietary restrictions limit food choices at restaurants, sporting events, and parties. Adults with PKU must consume more medical food than they did during childhood due to their size and protein requirements. Women with PKU must be on a severely restricted low-phenylalanine diet during pregnancy because hyperphenylalaninemia is a known teratogen (see text).
Inborn errors of metabolism are chronic diseases that are often treated by substantially modifying the diet. This can require considerable changes in lifestyle, precipitating financial and emotional hardships of which health-care providers need to be aware.
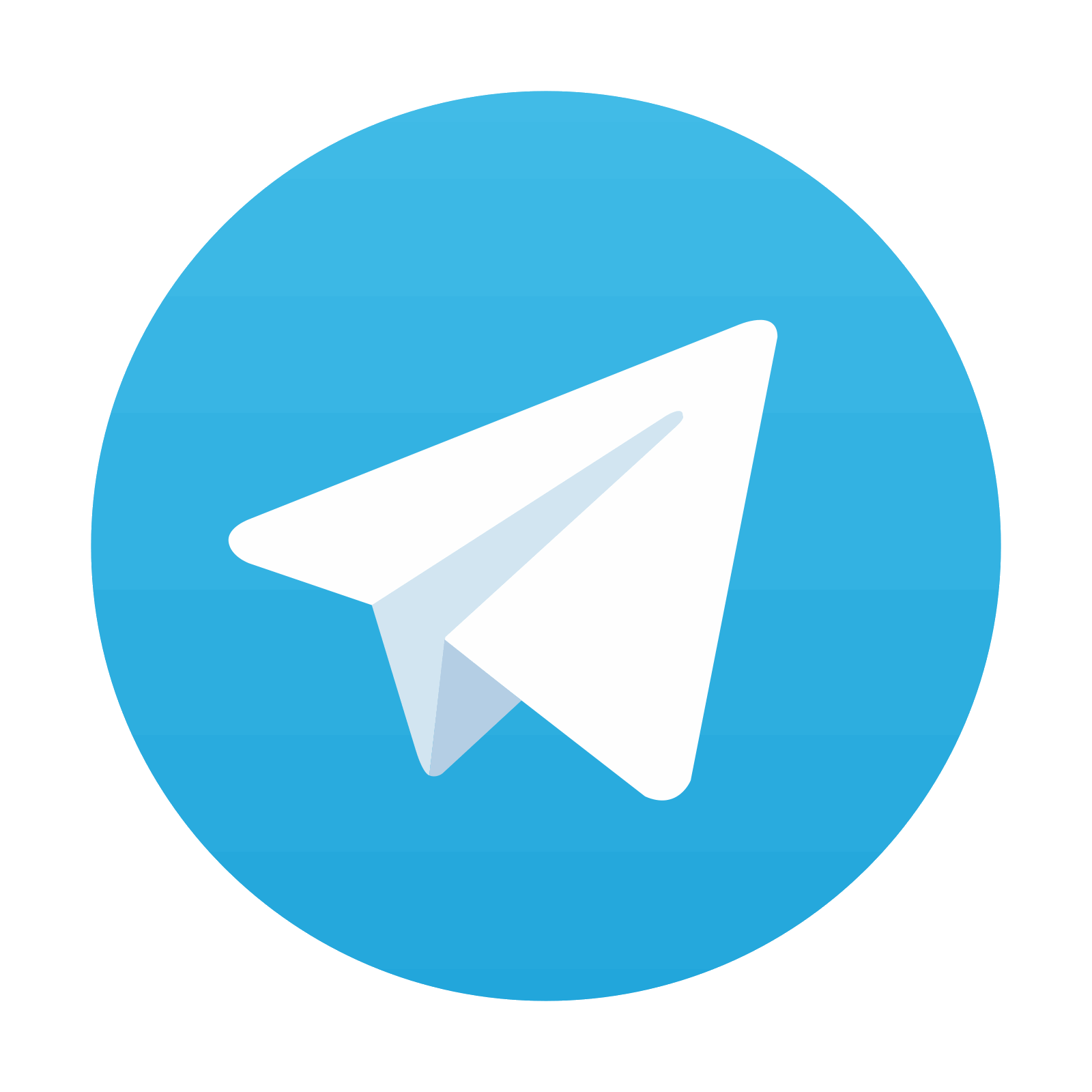
Stay updated, free articles. Join our Telegram channel
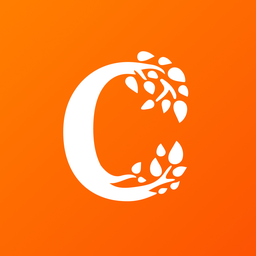
Full access? Get Clinical Tree
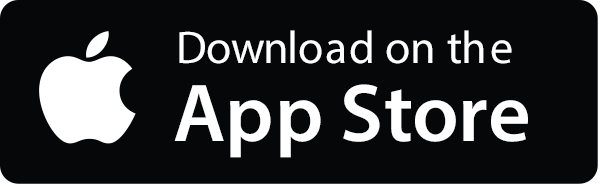
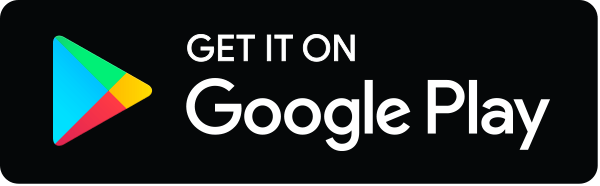
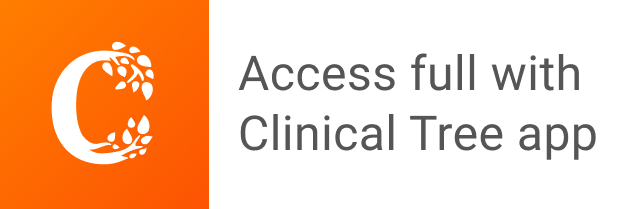