Fig. 7.1
Changes in auditory brain stem response (ABR) following a 106 dB, 2–20 kHz noise for 2 h in a mouse that had only a temporary threshold shift (TTS). The preexposure ABR (a) and 3 h (b) and 2 weeks (c) postexposure are shown. The animal exhibited a large TTS at 3 h (b) that recovered by 2 weeks (c)
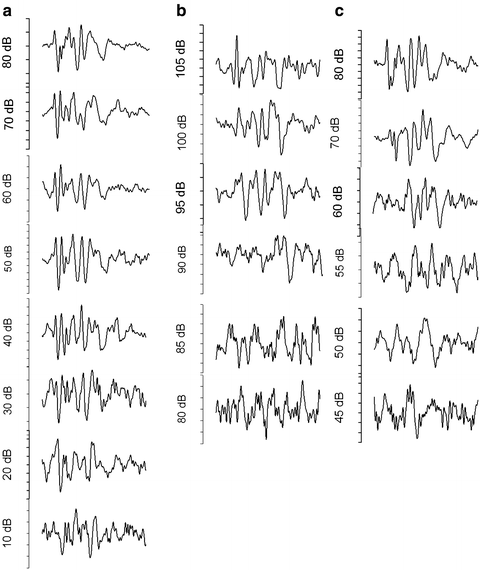
Fig. 7.2
Changes in auditory brain stem response (ABR) following a 106 dB, 2–20 kHz noise for 2 h in a mouse. While receiving the same noise exposure as the mouse in Fig. 7.2, this mouse showed a permanent threshold shift (PTS). The preexposure ABR (a), 3 h postexposure (b), and 2 weeks (c) postexposure are shown. The animal exhibited a large temporary threshold shift (TTS) at 3 h and (b) had a remaining PTS at 3 weeks following the exposure (c)
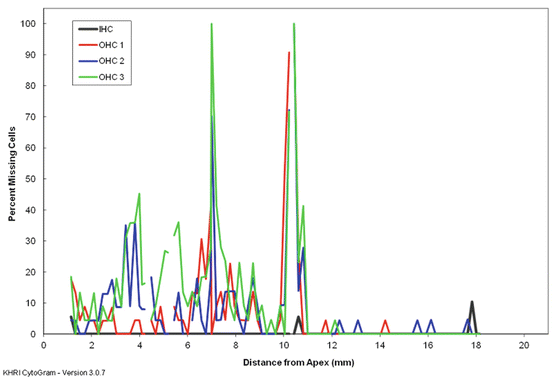
Fig. 7.3
A cytocochleogram from a Hartley guinea pig that received 122 dB, 4 kHz octave band noise for 5 h. Outer hair cell loss in the mid cochlea is associated with a permanent threshold shift
A wide variety of noises can cause a TTS, but generally a noise that induces a TTS will be mild to moderate in intensity and duration. Increasing either the intensity or duration of the sound can move a TTS-inducing noise into a PTS-inducing noise. Designing a “TTS” noise exposure involves finding the proper balance of intensity and duration to induce reversible changes, and one can increase either intensity or duration, and decrease the other, and still stay at a TTS-inducing level. Other characteristics, such as a broadband versus narrowband frequency exposure, and variation in the dynamic range or the “impulsive” nature of the sound exposure, will also influence whether a TTS or a PTS occurs. The spectral content of a sound can also influence the cochlear location of the TTS or PTS. Broadband noise will cause broad loss, while an intense pure tone will cause loss at ~1/2 octave above the pure tone frequency. There can often be both a TTS and a PTS component following a noise. By definition the TTS component is that which returns to normal levels, and the PTS component is what remains elevated. The mixed changes observed in the early post-noise period are in this case termed a compound threshold shift (Fig. 7.4).
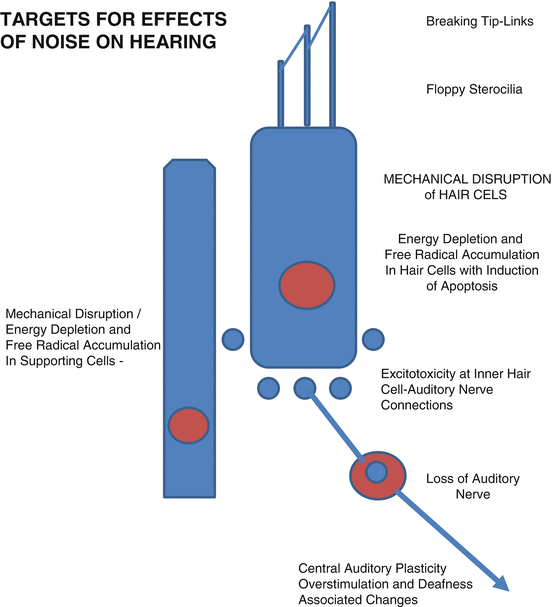
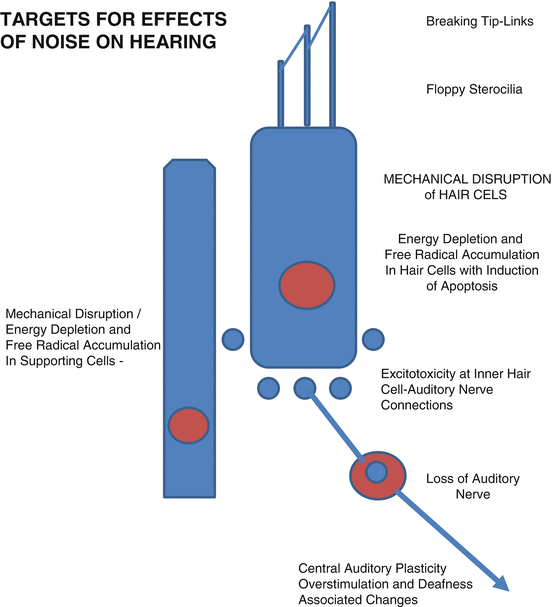
Fig. 7.4
Diagram showing some of the targets for the effects on noise that can cause hearing loss. From top to bottom, these are the tip-links of the inner or outer hair cell (inner hair cell is shown in schematic); hair cell stereocilia that can lose rigidity and become floppy; mechanical disruption, energy depletion, and free radical accumulation in hair cells that can induce apoptosis; mechanical disruption, energy depletion, and free radical accumulation in supporting cells that can disrupt their functions; excitotoxicity at inner hair cell-auditory nerve connections, loss of auditory nerve, and central auditory changes including overstimulation and deafness-induced plastic changes
7.1.1 Causes of TTS: General
While TTS is most often considered to reflect some underlying dysfunction, it can also be the result of an intrinsic protective mechanism, muting the effects of an intense sound that could otherwise cause greater damage and PTS. One such reflex targets the middle ear, using interneuron connections in the pons from auditory nuclei to both the motor nucleus of V and to the facial motor nucleus, with branches of the trigeminal and facial nerve then inducing contraction of the tensor tympani and the stapedius middle ear muscles, respectively (Borg and Counter 1989, for review). This causes a decrease in middle ear function for as long as the muscles are contracted, producing a “conduction deafness,” i.e., a TTS of about 20 dB. There are also medial efferent reflexes that can influence OHC motility and its amplification effect on the basilar membrane. This is a sound-evoked feedback system to the OHCs that reduces their amplification (Murugasu and Russell 1996; Russell and Murugasu 1997). This negative feedback gain control can reduce the effects of traumatizing noise stimulation (Rajan 2001a, b). However, this medial efferent feedback system does not contribute to the development of acquired resistance (Canlon et al. 1988) to acoustic trauma (Yamasoba and Dolan 1998). Efferent feedback also slows the effects of aging (Liberman et al. 2014). The middle ear muscles do not contribute to the development of acquired resistance (Ryan et al. 1994).
7.1.2 Causes of TTS: Hair Cells
As work by Hudspeth, Flock, Corey, and others (e.g., Hudspeth 1989; Flock and Orman 1983, Pickles and Corey 1992 for reviews) progressed to show the role of hair cell stereocilia in transduction, this also pointed to a role in TTS, with TTS appearing from a noise-induced dysfunction in stereocilia-related mechanisms (e.g., Canlon et al. 1987; Saunders et al. 1986a, b; Saunders and Flock 1986; Thorne et al. 1986). This generally falls under the category of “mechanical” causes. Tip-links between stereocilia were first identified by Pickles (Pickles et al. 1984) and then associated with the transduction channels (see Hackney and Furness 2013 for review). These tip-links appear to be very sensitive to noise overstimulation and can be lost with excessive stimulation (e.g., Pickles et al. 1987, Sakaguchi et al. 2009). If the tip-links are lost, then the transduction channel can no longer be opened. The loss of tip-links in OHCs diminishes the “amplifier” effect of outer hair cell motility; with tip-link loss there can be a 20–60 dB threshold shift. The loss of tip-links in IHCs reduces or prevents events leading to transmitter release and auditory nerve excitation, yielding an even greater threshold shift. The lost tip-links appear to be capable of rapid regeneration if and when the stereocilia maintain their rigidity and orientation (e.g., Zhao et al. 1996; Indzhykulian et al. 2013). Stereocilia have a specialized actin organization that is responsible for maintaining rigidity, and this actin organization can become disrupted (depolymerized) by a noise-induced mechanical stress (Tilney et al. 1982, Tilney and Saunders 1983). When this happens, the stereocilia become more flaccid (e.g., Canlon et al. 1987; Saunders et al. 1986a, b, Saunders and Flock 1986; Thorne et al. 1986) disrupting the transduction process. The actin organization can be regained once the noise stops. Restoration of stereocilia rigidity and orientation then allows for tip-links to be regenerated and relinked to the transduction channels. This whole process takes longer to occur than when only regeneration of tip-links is necessary, and therefore the loss of stereocilia rigidity generates a longer threshold shift. There is a tipping point for the mechanical forces where actual damage to stereocilia occurs, and this can move the threshold shift from temporary to permanent.
Other chapters in this volume will discuss in detail how different stresses, including noise, can induce different forms of oxidative stress in different cell types in the cochlea (and elsewhere) and cause either a temporary disruption of function or induce cell death pathways and permanent threshold shift when cells are lost. This chapter will therefore not go into detail in this area and will only give the broad general picture while referring to the chapters with additional information. However, we do want to reemphasize that TTS can also result when metabolic/intracellular changes including energy depletion and oxidative stress are below the level to initiate apoptotic pathways but sufficient to interfere with the function of cells in the cochlea. For OHCs this could impair transduction, depolarization, and other processes that drive OHC motility and the amplifier function derived from this. For IHCs this impairs transduction and the processes leading to transmitter release. Hair cells can be directly affected or can be affected by free radical-induced changes in other cellular elements, such as supporting cells.
7.1.3 Causes of TTS: Supporting Cells
While supporting cells were once believed to be predominantly passive and structural in their mature cochlear function, they are now known to play important active roles in homeostasis and in generating and maintaining the environment necessary for proper and efficient hair cell function (e.g., Wan et al. 2013, Monzack and Cunningham 2013). These dynamic supporting cell functions include generating and maintaining the proper ionic composition of cochlear fluids, generating and maintaining composition of various matrices and the reticular lamina, clearance of excess synaptic glutamate, managing ion diffusion, as well as more general waste management and “cleansing” across the cochlear environment. Supporting cells also secrete maintenance factors, such as NTF3, that can influence the status of hair cells, auditory neurons, and their connections. Recent studies show that supporting cells can secrete heat shock proteins upon stress which could then have a protective influence on hair cells (May et al. 2013).
Both noise-related mechanical stress and the noise-induced buildup of free radicals can disrupt supporting cell functions. It is not yet completely understood how disruption of supporting cells causes TTS. There can be buckling of supporting cells (predominantly pillar cells) in animals under noise exposure conditions that produce a TTS, and this could have the effect of decoupling stereocilia, and that would change basilar membrane mechanics and other cochlear dynamics (Nordmann et al. 2000; Harding and Bohne 2004). Interestingly, a wide variety of stresses, including noise and ototoxic drugs, have been found to induce both an earlier appearance and a higher level of free radicals in supporting cells compared to IHCs and OHCs (e.g., Henderson et al. 2006; Jiang et al. 2005; Ohinata et al. 2000, 2003; Yamashita et al. 2004). TTS can appear under conditions in which the predominant increase in free radicals occurs in supporting cells rather than hair cells. Despite this greater induction of free radicals in supporting cells compared to hair cells, it is rare to find the loss of supporting cells prior to the loss of hair cells. This suggests that supporting cells have a large potential for recovery and that the loss of supporting cell functions may jeopardize hair cells and their function. That the effects of noise on supporting cells may jeopardize hair recovery, leading to PTS, is discussed in additional detail later in this chapter.
7.2 Causes of TTS: Inner Hair Cell-Auditory Nerve Connections as a Target
The current definition of TTS as having no lasting harmful effects has now been challenged by recent studies from Kujawa and Liberman (2006, 2009). These studies found that even when a noise does not produce any loss of hair cells or permanent change in the sound-evoked ABR threshold, there can still be permanent neuronal changes and functional consequences which specifically include reduced suprathreshold ABR amplitude; i.e., the ABR is smaller at high sound levels, suggesting fewer neurons are responding to that sound. The overstimulation of the IHCs from noise or other stresses can cause excessive release of glutamate leading to excitotoxicity, with the swelling, bursting, and regression of some of the auditory nerve peripheral processes that had been connected to the IHC. Pujol and Puel (Puel et al. 1998; Pujol and Puel 1999) found that there is potential for lost processes to regenerate and reconnect to IHCs and suggested this might be a component of TTS. They also found recovery of suprathreshold response functions. The studies from Kujawa and Liberman (2006, 2009), however, show that such reconnection can be inefficient under their noise exposure conditions. Without reconnection there is eventual degradation and cell death of the auditory neuron. Moreover, the lost inner hair cell-auditory nerve (IHC-AN) connections tend to fall predominantly in one category of auditory nerve fibers, the low spontaneous rate group (Furman et al. 2013). Their loss then reduces the suprathreshold auditory brain stem responses. The apparent threshold may then recover, but there is a permanent loss of auditory neurons, and there might also be a reduced dynamic range. Regeneration and reconnection have been shown following noise overstimulation in guinea pig (Liu et al. 2012; Shi et al. 2013), and so auditory neuropathy following TTS noise may be variable depending on noise exposure conditions and species.
7.2.1 Strategies for Prevention and Repair
While many of the changes described above can occur independently, they can also occur simultaneously, with dominance depending on characteristics of the noise exposure. The timing for the different mechanisms and their effects can be different. One might expect that the most immediate effect would be from the mechanical influences of noise on the hair cell stereocilia and their tip-links which might in turn rapidly recover. Any effect through free radicals may depend on the cellular target (hair cell, supporting cells, auditory nerve, or other cochlear elements) and would be dependent on the rate of free radical formation and oxidative stress. It may be slower and longer lasting.
One immediate question is whether a true TTS should even be a target for prevention. Indeed, the two reflexes (middle ear and medial efferent) may have evolved for the purpose of providing a TTS for the purpose of protection from PTS. The loss of tip-links and/or loss of stereocilia stiffness could also provide valuable protection by stopping transduction and halting the progression from TTS to PTS. However, there are circumstances, such as for those serving in the military or in certain work situations, where even a brief temporary loss of hearing and communication can and often does put them at increased risk for survival (see reviews by McIlwaine 2009; Grantham 2011; Yankaskas 2013). Clearly, prevention of TTS could have value under the appropriate circumstance. In addition to the prevention of functional deficits, preventing the accumulation of free radicals in hair cells and/or supporting cells might also reduce free radical formation and TTS to a low level from which recovery is possible (TTS) instead of allowing free radical formation and TTS to proceed to a higher level where cell death occurs. Preventing excessive accumulation of free radical could not only prevent TTS but also prevent the progression from TTS to PTS. The TTS which results from accumulation of free radicals in hair cells and/or supporting cells is currently a major target for testing therapeutics. This is discussed in Chap. 9 by Le Prell and Lobarinas. The noise-induced loss of IHC-auditory nerve connections of low spontaneous rate auditory nerve fibers also points to a need for therapeutic interventions designed to prevent this loss. Perhaps anti-excitotoxicity agents, or agents that induce a more efficient regeneration or reconnection, will be the most effective drugs for this purpose.
7.2.2 Noise-Induced Permanent Threshold Shift
Noise-induced permanent threshold shifts are generally associated with either cell death or with a permanent loss of cell function in remaining cells. The dysfunctional cells can be destined for cell death at a later time, even if present at the time of assessment, but can also remain in a dysfunctional state for long periods of time or even indefinitely. While a noise-induced cell loss causing permanent threshold shifts is most often “sensorineural” from the loss of hair cells and/or loss of auditory nerve, there can also be a loss of non-sensorineural elements. For example, noise can cause the loss of type IV fibroblasts that influence cochlear mechanical processes, and their loss can therefore result in less efficient transduction and threshold shifts (Adams 2009; Chap. 3 by Ohlemiller) without any loss of hair cells or auditory nerve. Sensorineural loss can be secondary to “intermediate steps” involving cell death in other cochlear elements including lateral wall and stria that can lead to changes (e.g., in endolymphatic potential or ionic transport) that can induce indirect loss of hair cells or auditory nerve (Chap. 3). However, the authors are not aware of any reports of finding a noise-induced loss of supporting cells in the organ of Corti without any adjacent loss of hair cells, despite supporting cells generally being the first site of free radical formation following stresses such as noise, ototoxins, and hypoxia.
7.3 Mechanisms Underlying Cell Death
Noise-induced cell death can be from apoptosis, a programmed cell death under the control of specific intracellular molecular pathways (see Chap. 19), or from necrosis, a more passive, less controlled mode (Yang et al. 2004). Necrosis is most often associated with mechanical damage to or within cells resulting in the cell or cellular elements swelling, breaking up, or rupturing. An inflammatory response is often associated with necrosis. There is often considered to be a “critical level” for noise exposures above which mechanical damage begins (Henderson et al. 1994; Henderson and Hamernik 2011, Wang et al. 2002). The critical level can be influenced by multiple factors including the length of time of the noise exposure, the intensity of the noise exposure, and the type of noise (e.g., continuous versus impulse).
The same exposure intensity/duration can cause hearing loss in one subject and not in another similar subject, both in people and animal models, a topic explored in detail by Spankovich in Chap. 6. The amount of hearing loss can have large variability among subjects. There could be genetic or epigenetic effects through multiple genes that directly or indirectly influence sensitivity and susceptibility (see Chaps. 8, 14, and 17). These could influence the strength/efficiency of endogenous protective pathways such as the heat shock proteins or the levels of endogenous antioxidants. Variations, including heat shock protein-associated genes, have been found in genotyping studies comparing human populations with and without hearing loss (Konings et al. 2009; Sliwinska-Kowalska and Davis 2012, Sliwinska-Kowalska and Pawelczyk 2013).
Preconditioning or “toughening” can also come into play where previous low-level, non-damaging noise exposure(s) or other previous stresses to the cochlea can protect from a later potentially more damaging noise exposure (see Niu and Canlon 2002 for review). While a role for the middle ear or medial efferent reflexes has been ruled out (Ryan et al. 1994; Yamasoba and Dolan 1998), other endogenous protective mechanisms may be involved. Preconditioning may increase resistance to noise-induced oxidative stress by effects on endogenous levels of free radical scavengers/antioxidants (e.g., Harris et al. 2006, Yamasoba et al. 1998). It may also involve other stress pathways such as those involving heat shock proteins. Prior induction of heat shock proteins has been shown to provide protection from noise (Yoshida et al. 1999), and a low-level noise preconditioning will upregulate heat shock proteins and provide protection from an aminoglycoside ototoxicity (Roy et al. 2013). Conditioning may also be generated from stress pathways of the hypothalamic-pituitary-adrenal axis, involving glucocorticoid receptors and corticosteroids (Tahera et al. 2007). Other potential factors include changes in the presynaptic region of hair cells (Canlon et al. 1993), alterations in F-actin distribution in supporting cells and hair cells (Hu and Henderson 1997), and a decrease in calbindin D-28 immunoreactivity in OHCs (Canlon 1996).
Mechanical forces from the noise exposure can act at an individual cell level but can also act on the general structure of the cochlea. Mechanical stress can result in the breaking of junctions separating cochlear fluids and induce cell death in the region of leakage by ionic poisoning of cells (e.g., Ahmad et al. 2003). Mechanical disruption of individual cells can range from bursting and rupturing of entire cells to effects that are limited to specific cellular or intracellular components, such as F-actin in the cuticular plate (Hu and Henderson 1997). For hair cells, the stereocilia are particularly sensitive to noise, and there can be a gradient in the effect and damage to the stereocilia depending on the force and duration of the noise. At the low end of the gradient is the loss of tip-links and actin structure that can recover, giving TTS, next would be a permanent loss of microstructure organization leading to dysfunction and cell death by apoptosis or active removal by scar formation, greatest would be a physical loss and rupture leading to hair cell death necrosis.
There appears to be a more limited ability for cochlear hair cells to repair themselves compared to hair cells in vestibular end-organs. One potential explanation is “trigger-happy supporting cells” eager to begin the process of scar formation as a protective mechanism. Raphael et al. (1996) suggested that supporting cells are programmed to maintain the barrier between endolymph and perilymph and can respond to cues (or lack of cues) from hair cells that have some level of damage or dysfunction. The surrounding supporting cells respond by forming a new tight junction, the scar, in the process choking and killing the noise-damaged hair cell before it has a chance to repair and recover. More often, scar formation is part of an orderly program as a hair cell goes through the controlled progression of cell death following one of several intracellular molecular pathways during apoptosis.
Noise-induced cell death by apoptosis is most often the consequence of stress-induced processes that can be induced by free radical formation/oxidative stress and/or energy depletion/mitochondrial changes. There is a gradation/continuum in effect depending on the amount, type, and duration of noise stress and resulting amount of free radical formation and energy depletion. There can be immediate and delayed effects. Studies have shown free radical formation beginning during noise exposure or several days after the noise exposure (Yamashita et al. 2004). Histopathology studies show that the margins of the region of cell death continue to expand well after the noise exposure (Hu 2012 for review). This suggests different mechanisms and interactions among cellular elements (see Sect. 7.3.1) and also influences approaches for therapeutics for prevention and repair.
7.3.1 Role of Supporting Cells in PTS
Studies examining free radical formation following noise in the organ of Corti show that cells of the lateral wall and then supporting cells are among the first cochlear elements to demonstrate increases in free radicals following the noise overstimulation and these non-sensory cells also often show the highest levels of free radical increases (Chap. 3; see also Yamashita et al. 2004). Nonetheless, hair cells can move to noise-induced apoptosis even when supporting cells do not, suggesting that the threshold to induce apoptotic pathways is lower in hair cells. Supporting cells may also be more capable of repair or using endogenous protective pathways to block apoptosis. Even if apoptosis does not manifest in the supporting cells, free radical formation and energy depletion can decrease their function. Supporting cells have an active role in generating and maintaining the environment for efficient transduction as well to support the health of other cochlear elements (Wan et al. 2013, Monzack and Cunningham 2013), with their junctional complexes essential for K+ transport and homeostasis. Less efficient supporting cell function following noise could compromise the ability of hair cells to withstand stress and compound the direct effect of noise of hair cells. It could also explain the later occurring effects on hair cells, if supporting cells go through a period where they are less efficient at maintaining the cochlear environment.
7.3.2 Approaches for Protection: Therapies
Mechanical changes offer less opportunity for pharmacological therapeutic interventions for protection or repair, although the heat shock protein pathway can act against protein folding and enhancing this pathway could protect or repair such changes. When mechanical stress induces apoptosis, the pathway can be blocked (e.g., Bielefeld et al. 2011; Fetoni et al. 2014). Indeed, apoptosis offers the greatest opportunity for pharmacological interventions, and this has been the topic of multiple recent reviews, i.e., Abi-Hachem et al. (2010) and Poirrer et al. (2010). Protection could be achieved by reducing the initiating (upstream) factors, directly neutralizing free radicals through the use of free radical scavengers, or by reducing oxidative stress and/or energy depletion by enhancing intracellular protective pathways. Another approach is by negating or interrupting the cell death pathways. These are discussed in depth in Chap. 20 by Van de Water et al. Because there can be delays in free radical formation and apoptosis following noise (Yamashita et al. 2004), there is a window for protection from these processes during a period of days following noise, as well as opportunity to enhance repair (e.g., Yamashita et al. 2005). These approaches are relevant to drug-induced hearing loss (Chaps. 10 and 11), age-related hearing loss (Chap. 16), and hearing loss due to surgical trauma (Chap. 19).
7.4 Noise-Induced Effects on Central Auditory Pathways
Noise overstimulation will influence the mature central auditory pathways in several ways. There can be an immediate synaptic overstimulation from the noise. With glutamate as the excitatory transmitter in many central auditory synapses, there is a potential for excitotoxicity from this initial overstimulation. Evidence of excitotoxicity, however, is rarely found except in animal models such as gerbil where the formation of microcysts in the cochlear nucleus is influenced by ambient levels of noise (Statler et al. 1990). There are also central auditory changes that are part of the “normal” response to noise-induced increases in the level of auditory activity that are part of “active listening” (Fritz et al. 2005; Zion Golumbic et al. 2012 for reviews) and experience-associated auditory plasticity (Schreiner and Polley 2014 for review). Even as the noise overstimulation continues, the increased activity in the central pathways may be of short duration because of TTS- or PTS-related changes in the cochlea that will diminish the activity in the auditory nerve and reduce the overstimulation, often to under-stimulation. The duration of the under-stimulation or “deafness” would be related to the duration of the TTS or appearance of PTS. The under-simulation or deafness may only be across a restricted frequency range, with tonotopic regions and divisions influenced in different ways. Deafness-associated changes might then be expected to occur.
There is a large literature on deafness-associated changes to the central auditory pathways. These can be brief and reversible or permanent, depending on the characteristics of the noise overstimulation and its effects in the cochlea. As with overstimulation, deafness can induce central auditory changes that are part of the normal sound processing in active listening and experience-dependent plasticity. Depending on the type and duration of the noise-induced deafness, these central auditory changes may not reverse in the “normal” fashion and may persist and induce other changes that are not normally occurring. Such “maladaptive changes” may contribute to the generation of tinnitus (Wang et al. 2011a, b for review) as discussed later in this section. Deafness-associated central auditory changes can involve up- and downregulation of neurotransmitters, receptor subunits, ion channels, and other synaptic elements that change the balance between excitation and inhibition as well as neuronal excitability and intrinsic properties (see Altschuler et al. 2004; Caspary et al. 2008; Dong et al. 2009 for reviews). Changes in the inhibitory amino acids GABA and glycine and in GABA receptor subunits appear to be common deafness-induced changes (Caspary et al. 2008; Dong et al. 2009, Asako et al. 2005; Buras et al. 2006). There can also be deafness-associated changes in glutamate receptors (Sato et al. 2000 for review). Ion channels can change phosphorylation state as a consequence of changes in auditory activity (Brown and Kaczmarek 2011). Many ion channels have deafness-associated changes in expression including the two-pore domain potassium channels (Holt et al. 2006; Cui et al. 2007). The degree of deafness required to produce central auditory changes may vary for each different type of change.
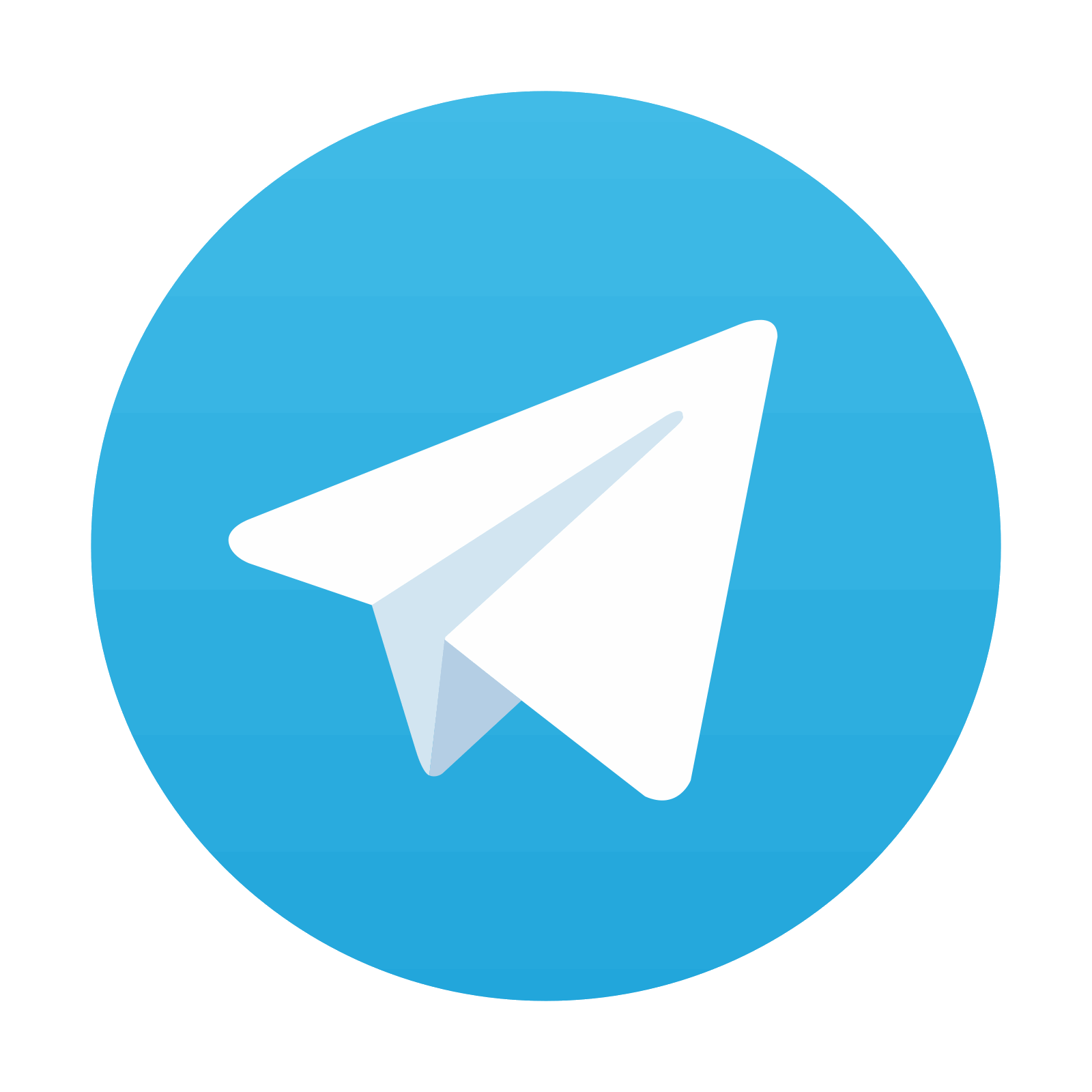
Stay updated, free articles. Join our Telegram channel
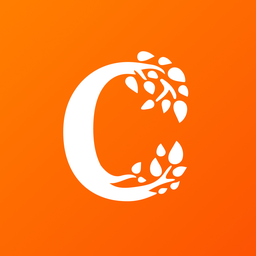
Full access? Get Clinical Tree
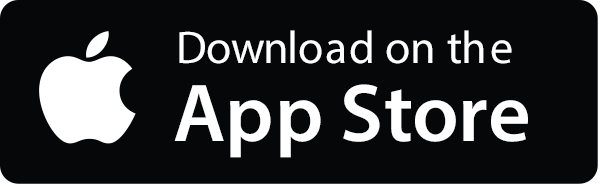
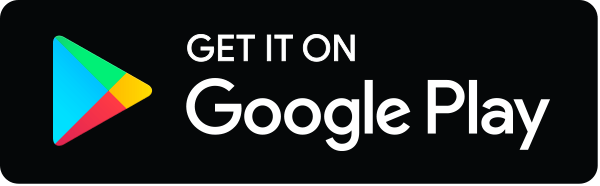