Figure 12-1. Symptoms of ADHD. There are three major categories of symptoms associated with attention deficit hyperactivity disorder (ADHD): inattention, hyperactivity, and impulsivity. Inattention itself can be divided into difficulty with selective attention and difficulty with sustained attention and problem solving.
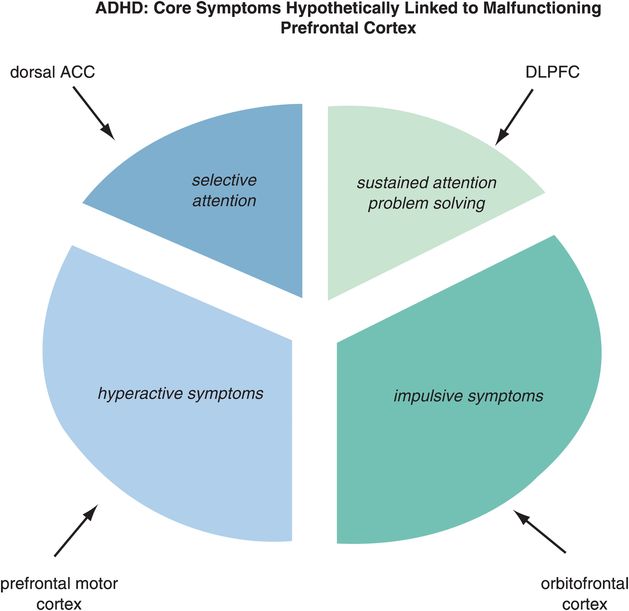
Figure 12-2. Matching ADHD symptoms to circuits. Problems with selective attention are believed to be linked to inefficient information processing in the dorsal anterior cingulate cortex (dACC), while problems with sustained attention are linked to inefficient information processing in the dorsolateral prefrontal cortex (DLPFC). Hyperactivity may be modulated by the prefrontal motor cortex and impulsivity by the orbitofrontal cortex (OFC).
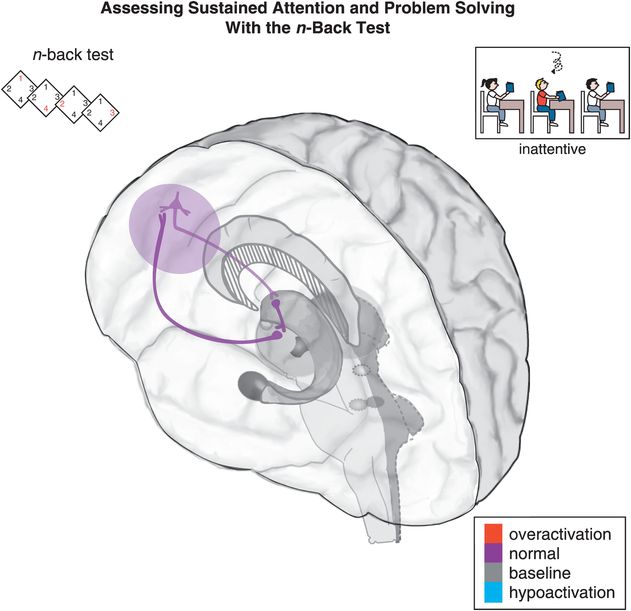
Figure 12-3. Sustained attention and problem solving: the n-back test. Sustained attention is hypothetically modulated by a cortico-striato-thalamo-cortical (CSTC) loop that involves the dorsolateral prefrontal cortex (DLPFC) projecting to the striatal complex. Inefficient activation of the DLPFC can lead to difficulty following through or finishing tasks, disorganization, and trouble sustaining mental effort. Tasks such as the n-back test are used to measure sustained attention and problem-solving abilities. In the 0-back variant of the n-back test, a participant looks at a number on the screen, and presses a button to indicate which number it is. In the 1-back variant, a participant only looks at the first number, and when the second number appears the participant is supposed to press a button corresponding to the first number. Higher n values are correlated with increased difficulty in the test.
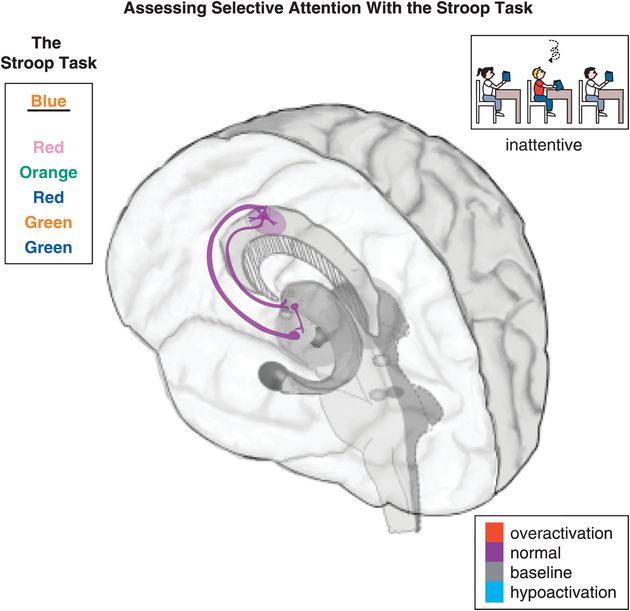
Figure 12-4. Selective attention: the Stroop task. Selective attention is hypothetically modulated by a cortico-striato-thalamo-cortical (CSTC) loop arising from the dorsal anterior cingulate cortex (dACC) and projecting to the striatal complex, then the thalamus, and back to the dACC. Inefficient activation of dACC can result in symptoms such as paying little attention to detail, making careless mistakes, not listening, losing things, being distracted, and forgetting things. An example of a test that involves selective attention, and thus should activate the dACC, is the Stroop task. The Stroop task requires the participants to name the color with which a word is written, instead of saying the word itself. In the present case, for example, the word “blue” is written in orange. The correct answer is therefore “orange,” while “blue” is the incorrect choice.
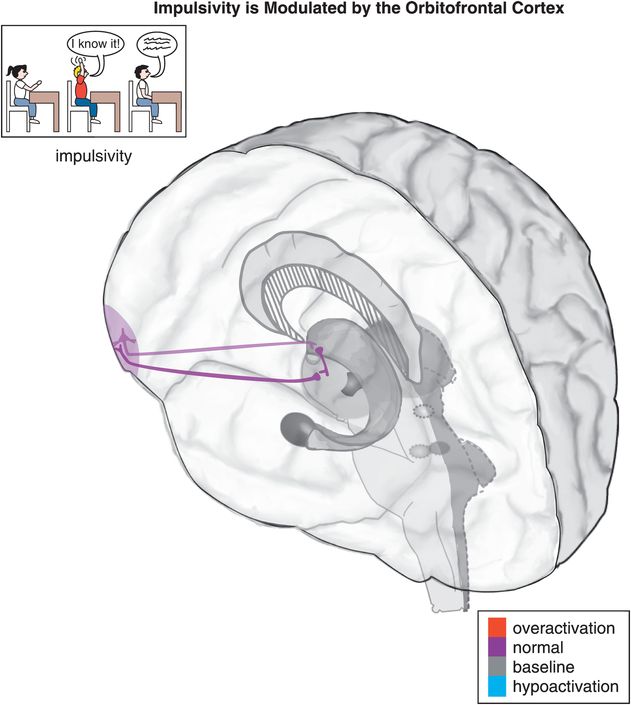
Figure 12-5. Impulsivity. Impulsivity is associated with a cortico-striato-thalamo-cortical (CSTC) loop that involves the orbitofrontal cortex (OFC), the striatal complex, and the thalamus. Examples of impulsive symptoms in ADHD include talking excessively, blurting things out, not waiting one’s turn, and interrupting.
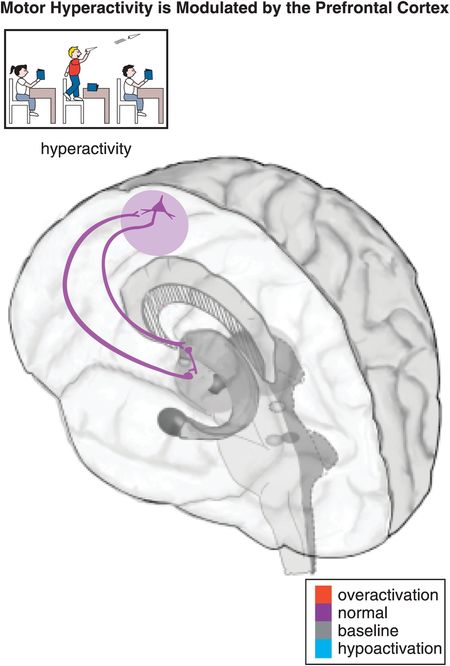
Figure 12-6. Hyperactivity. Motor activity, such as hyperactivity and psychomotor agitation or retardation, can be modulated by a cortico-striato-thalamo-cortical (CSTC) loop from the prefrontal motor cortex to the putamen (lateral striatum) to the thalamus and back to the prefrontal motor cortex. Common symptoms of hyperactivity in children with ADHD include fidgeting, leaving one’s seat, running/climbing, being constantly on the go, and having trouble playing quietly.
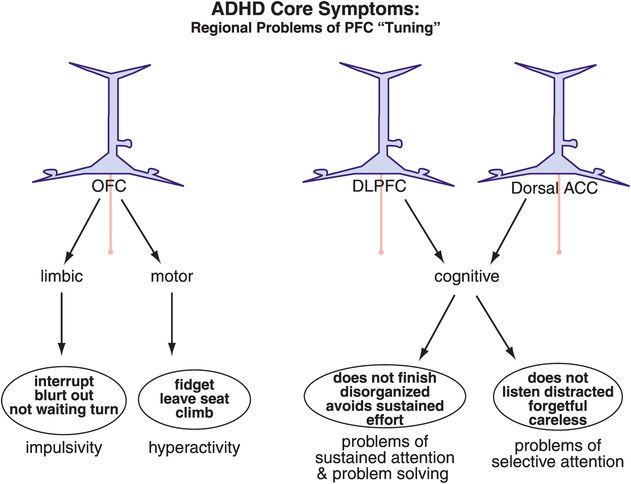
Figure 12-7. ADHD: out-of-tune prefrontal cortex. Different brain areas are hypothetically important in the symptoms of ADHD. Alterations within the orbitofrontal cortex (OFC) are hypothesized to lead to problems with impulsivity or hyperactivity. Inadequate tuning of the DLPFC or the dACC can respectively lead to sustained or selective attentive symptoms. It is becoming increasingly clear that dysfunction in specific brain areas leads to specific symptoms, such that abnormalities in the orbitofrontal–limbic motivation networks have been observed in children with conduct disorder, while aberrations in the dorsolateral cognitive network have been observed in children with problems of sustained attention.
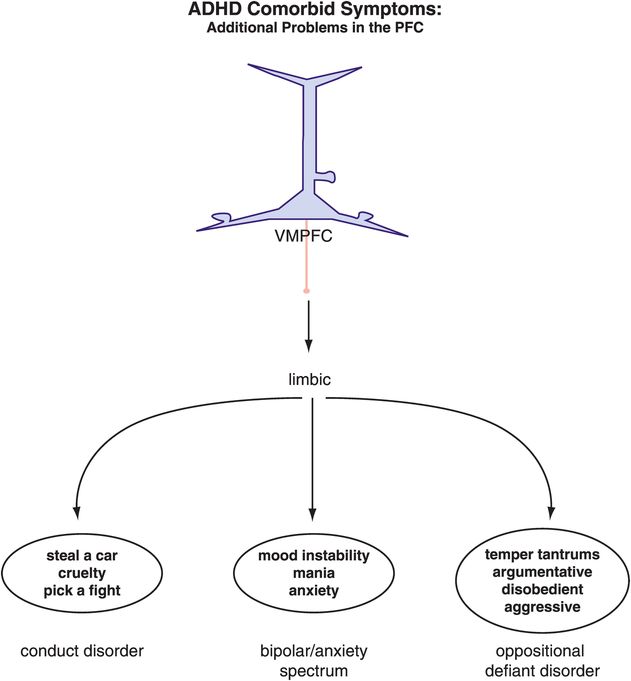
Figure 12-8. ADHD and comorbid symptoms. The comorbidities associated with ADHD are often the result of similar or additional dysfunctions within the prefrontal cortex–limbic network. Many mood disorders are comorbid with ADHD both in children and in adults, and it has been suggested that the symptoms in adults might be most disabling if the comorbidities were already present in the child. This emphasizes the importance of treating all the symptoms in the younger population of ADHD patients in order to maximize their chances of a “regular” adult life. VMPFC, ventromedial prefrontal cortex.
Another symptom of ADHD is selective inattention, or not being able to focus, and thus differing from the executive dysfunction described above. The symptom of difficulty focusing is hypothetically linked to inefficient information processing in a different brain area, namely the dorsal anterior cingulate cortex (dACC) (Figures 12-2, 12-4, 12-7). The dACC can be activated by tests of selective attention, such as the Stroop test (explained in Figure 12-4). ADHD patients may either fail to activate the dACC when they should be focusing their attention, or they activate this part of the brain very inefficiently and only with great effort and easy fatigability.
Other areas of prefrontal cortex that are hypothetically not functioning efficiently in ADHD are the orbitofrontal cortex (OFC), linked to symptoms of impulsivity (Figures 12-2, 12-5, 12-7) and the supplementary motor area, linked to symptoms of motor hyperactivity (Figures 12-2, 12-6, 12-7). The OFC is hypothetically linked to a wide variety of symptoms that cut across several psychiatric conditions, including impulsivity in ADHD (Figures 12-2, 12-5, 12-7), impulsivity and violence in schizophrenia (discussed in Chapter 4), suicidality in depression (discussed in Chapter 6), impulsivity in mania (discussed in Chapter 6), and impulsivity/compulsivity in substance abuse (discussed in Chapter 14). Impulsive symptoms in other psychiatric conditions commonly comorbid with ADHD are also hypothetically related to the orbitofrontal cortex, such as conduct disorder, oppositional defiant disorder, and bipolar disorder (Figure 12-8). Impulsivity is discussed extensively in Chapter 14 (see Tables 14-1 through 14-8 and Figures 14-1 through 14-5).
ADHD as a disorder of inefficient “tuning” of the prefrontal cortex by dopamine and norepinephrine
ADHD patients generally cannot activate prefrontal cortex areas appropriately in response to cognitive tasks of attention and executive functioning. Some studies suggest that this is because dopamine (DA) and norepinephrine (NE) dysregulation in ADHD prevents the normal “tuning” of pyramidal neurons in the prefrontal cortex. In the case of DA and NE neurons, their normal firing at baseline is considered slow and “tonic,” stimulating a few receptors on postsynaptic neurons and allowing for optimal signal transmission and downstream neuronal firing (Figure 12-9). Modest levels of NE release will hypothetically improve prefrontal cortical function by stimulating postsynaptic α2A receptors, but high levels of NE release will lead to impaired working memory when α1 and β1 receptors are also recruited (Figure 12-9). Similarly, modest levels of DA will first stimulate D3 receptors, as these are more sensitive to DA than D1 or D2 receptors (Figure 12-9). Hypothetically, low to moderate, but not high, levels of D1 receptor stimulation is beneficial to optimizing prefrontal cortical functioning.
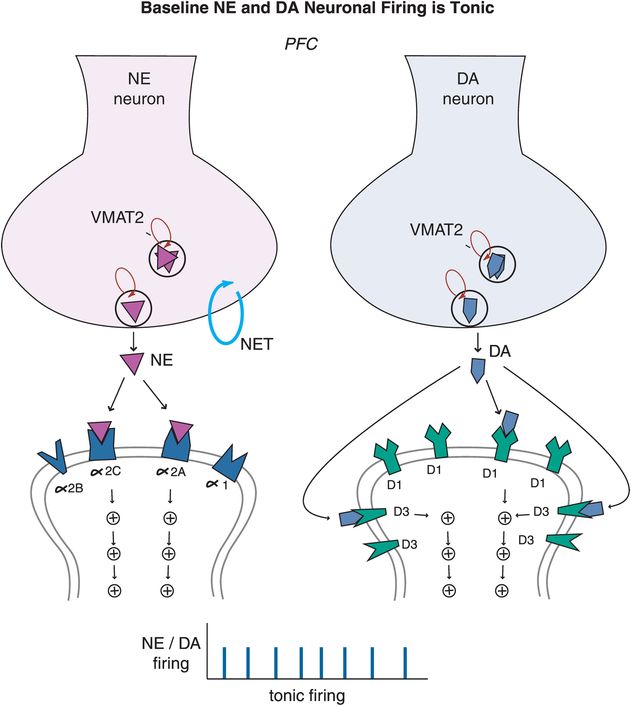
Figure 12-9. Baseline tonic firing. Modulation of prefrontal cortical function, and therefore regulation of attention and behavior, relies on the optimum release of dopamine (DA) and norepinephrine (NE). Under normal conditions, released NE and DA in the prefrontal cortex stimulate a few receptors on postsynaptic neurons allowing for optimal signal transmission and neuronal firing. At modest levels, NE can improve prefrontal cortical function by stimulating postsynaptic α2A receptors, but will lead to impaired working memory at high levels when α1 and β1 receptors are also recruited. Similarly, modest levels of DA will first stimulate D3 receptors as these are more sensitive to DA than D1/D2 receptors. Low to moderate, but not high, levels of D1 receptor stimulation can be beneficial to prefrontal cortical functioning. In the case of both DA and NE systems, moderation is certainly key.
Dopamine neurons in particular can also exhibit bursts of firing, called phasic (Figure 12-10). Phasic DA release is thought to reinforce learning and reward conditioning, providing the motivation to pursue naturally rewarding experiences such as education, recognition, career development, enriching social and family connections, etc. When the phasic DA system is hijacked by drugs, it can induce uncontrolled DA firing that reinforces the reward of drug abuse, and lead to compulsive behaviors such as mindless self-destructive drug seeking (discussed in Chapter 14). Thus, finely tuning the DA reward pathway in the nucleus accumbens and its connections to the amygdala and prefrontal cortex by attaining a low level of phasic firing in relation to tonic firing will theoretically lead to proper functioning of this complex system.
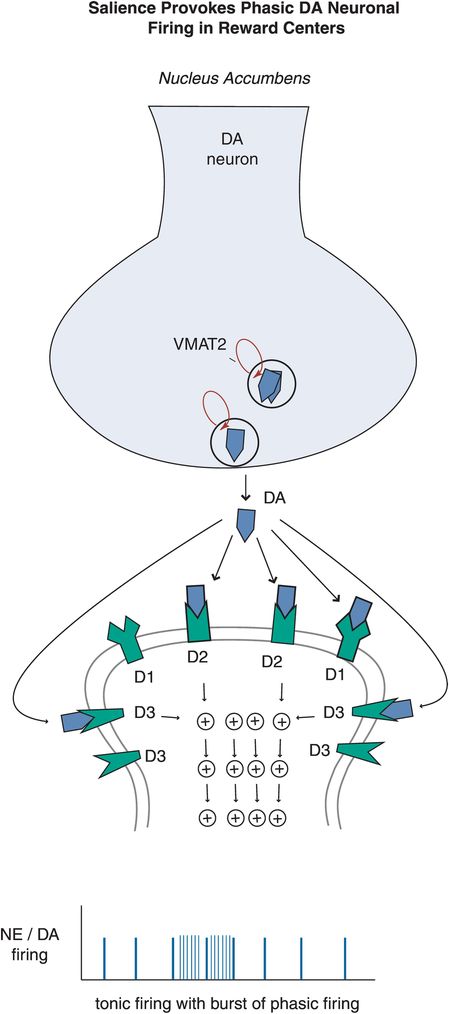
Figure 12-10. Salience-provoked phasic firing. While tonic firing, as seen in the prefrontal cortex, is often preferred in neuronal systems, a little bit of phasic firing of DA neurons in the nucleus accumbens can be a good thing. Phasic firing will lead to bursts of DA release, and when this happens in a controlled manner it can reinforce learning and reward conditioning, which can provide the motivation to pursue naturally rewarding experiences (e.g., education, career development, etc). When this system however is out of bounds, it can induce uncontrolled DA firing that reinforces the reward of taking drugs of abuse, for example, in which case the reward circuitry can be hijacked and impulses are followed by the development of uncontrolled compulsions to seek drugs.
In ADHD, imbalances in NE and DA circuits in the prefrontal cortex hypothetically cause inefficient information processing in prefrontal circuits, and thus the symptoms of ADHD (as shown for circuits in Figures 12-2 through 12-8). At the level of NE and DA synapses in the prefrontal cortex, deficient signaling in prefrontal cortical DA and NE pathways is reflected by decreased neurotransmission and thus reduced stimulation of postsynaptic receptors (Figure 12-11). Agents that can lead to increased release of these two neurotransmitters or increased tonic firing of these neurons will be hypothetically beneficial in patients with ADHD by bringing prefrontal activity back to optimal levels. On the other hand, ADHD can also be hypothetically associated with excessive signaling in prefrontal cortical DA and NE pathways, particularly in adolescents and adults (Figure 12-12). That is, stress can activate NE and DA circuits in the prefrontal cortex, leading to high levels of DA and NE release, and thus cause an excess of phasic NE and DA firing (Figure 12-12). This excessive NE and DA neurotransmission may be the underpinning of the development of drug and alcohol abuse, impulsivity, inattention and anxiety, all comorbid with ADHD, particularly in adolescents and adults.
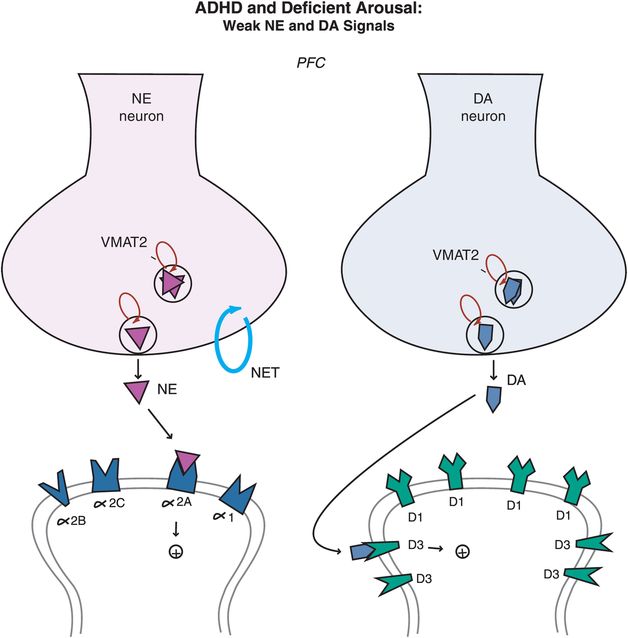
Figure 12-11. ADHD and deficient arousal. Besides being a key player in the arousal pathways, the prefrontal cortex is also the main brain area where imbalances in NE and DA systems hypothetically occur in ADHD. Deficient signaling in prefrontal cortical DA and NE pathways is reflected by reduced stimulation of postsynaptic receptors. Agents that can lead to (1) increased release of these two neurotransmitters, or (2) increased tonic firing of these neurons, will be hypothetically beneficial in patients with ADHD by bringing prefrontal activity back to optimal level.
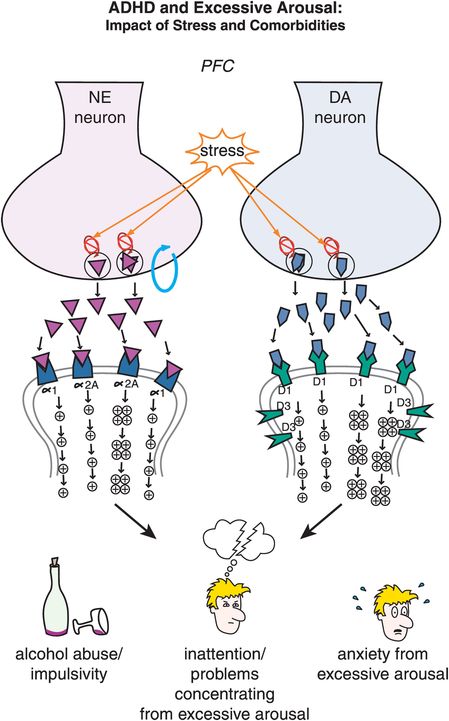
Figure 12-12. ADHD and excessive arousal. Nontreated adults with ADHD can often be stressed as they are trying to deal with their disorder while at the same time attempting to accomplish as much as their peers. Unfortunately, stress can activate NE and DA circuits in the prefrontal cortex, leading to an excess of phasic NE and DA firing. This excessive NE and DA neurotransmission may herald the development of impulsivity, inattention, and comorbidities associated with ADHD such as anxiety and substance abuse. This emphasizes the notion that treatment of all comorbid disorders is necessary to attain good patient outcomes.
So, is the prefrontal cortex out of tune when NE and DA are too high or too low? The answer seems to be that either too much or too little stimulation by NE or DA can cause inefficient information processing, because for the prefrontal cortex to work properly, cortical pyramidal neurons need to be tuned, meaning that moderate stimulation of α2A receptors by NE and D1 receptors by DA is required, neither too high nor too low. In theory, the role of NE is to increase the incoming signal by allowing for increased connectivity of the prefrontal networks, while the role of DA is to decrease the noise by preventing inappropriate connections from taking place. Pyramidal cell function is optimal at the top of this inverted U-shaped curve, when stimulation of both α2A and D1 receptors is moderate (Figure 12-13). If stimulation at α2A and D1 receptors is too low (left side of Figure 12-13), all incoming signals are the same, preventing a person from focusing on one single task (unguided attention). When stimulation is too high (right side of Figure 12-13) the signals get scrambled as additional receptors are recruited, again misguiding a person’s attention. A balanced, moderate stimulation of α2A and D1 receptors is thus critical for correct interpretation of an incoming signal.
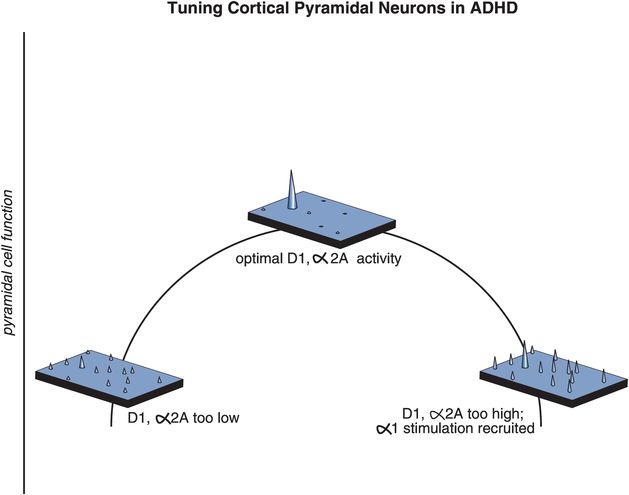
Figure 12-13. ADHD and maladaptive signal-to-noise ratios. In order for the prefrontal cortex to work properly, moderate stimulation of α2 receptors by NE and D1 receptors by DA is required. In theory, the role of NE is to increase the incoming signal by allowing for increased connectivity of the prefrontal networks, while the role of DA is to decrease the noise by preventing inappropriate connections from taking place. At the top of the inverted U-shaped curve depicted here, stimulation of both α2A and D1 receptors is moderate and pyramidal cell function is optimal. If stimulation at α2A and D1 receptors is too low (left side), all incoming signals are the same, making it difficult for a person to focus on one single task (unguided attention). If stimulation is too high (right side), incoming signals get jumbled as additional receptors are recruited, resulting in the misdirection of attention.
In prefrontal cortex, α2A and D1 receptors are often located on the spines of cortical pyramidal neurons, and can thus gate incoming signals (Figures 12-14 through 12-18). Alpha-2A receptors are linked to the molecule cyclic adenosine monophosphate (cAMP) via the inhibitory G protein, or Gi (Figure 12-14). D1 receptors, on the other hand, are linked to the cAMP signaling system via the stimulatory G protein (Gs) (Figure 12-14). In either case, the cAMP molecule links the receptors to the hyperpolarization-activated cyclic nucleotide-gated (HCN) cation channels. An open channel will lead to a low membrane resistance, thus shunting inputs out of the spine. In the presence of an open channel, the signal leaks out and is therefore lost. However, when these channels are closed, the incoming signal survives and can be directed down the neuron to strengthen the network connectivity of similar neurons and lead to the appropriate signal and response.
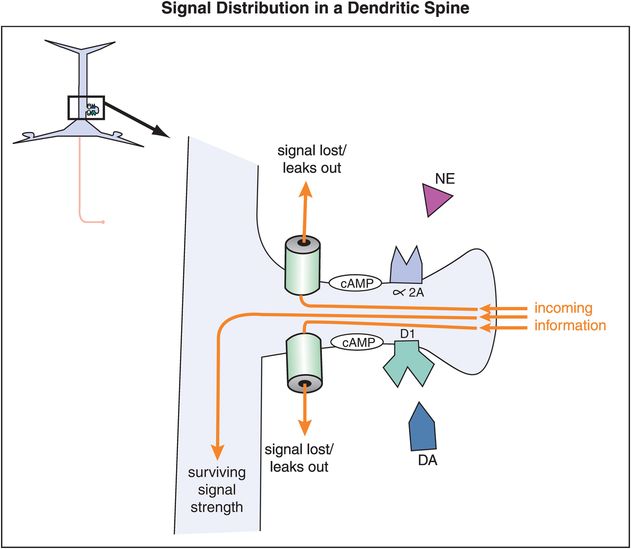
Figure 12-14. Signal distribution in a dendritic spine. The location of α2A and D1 receptors on dendritic spines of cortical pyramidal neurons in the prefrontal cortex allows them to gate incoming signals. Both α2A and D1 receptors are linked to the molecule cyclic adenosine monophosphate (cAMP). The effects on cAMP from NE and DA binding at their respective receptors are opposite (inhibitory in the case of NE and excitatory in the case of DA). In either case the cAMP molecule links the receptors to the hyperpolarization-activated cyclic nucleotide-gated (HCN) cation channels. When HCN channels are open, incoming signals leak out before they can be passed along. However, when these channels are closed, the incoming signal survives and can be directed down the neuron.
When NE, or a noradrenergic agonist, binds to an α2A receptor, the activated Gi-linked system inhibits cAMP, thereby closing the HCN channel (Figure 12-15). Closure of the channel allows the signal to go through the spine and down the neuron, thereby strengthening network connectivity with similar neurons (Figure 12-15). So in general, in the prefrontal cortex, stimulation of α2A receptors strengthens an incoming signal.
By contrast, stimulation of D1 receptors leads to weakening of the signal (Figure 12-16). That is, when DA, or a DA agonist, binds to a D1 receptor, the activated Gs-linked system will lead to increased stimulation – or opening – of HCN channels. The opening of the HCN channels, especially if excessive, will lead to leakage of the signal, thereby shunting any input out of the spine. So excessive stimulation of D1 receptors will, in contrast to stimulation of α2A receptors, result in the dissipation and/or weakening of a signal. The mechanism of action of α2A (Figure 12-15) and D1 receptors (Figure 12-16) explains in general why moderate stimulation of both types of receptors (Figure 12-14) is preferred in order to strengthen the signal-to-noise ratio in prefrontal cortical neurons (Figure 12-17).
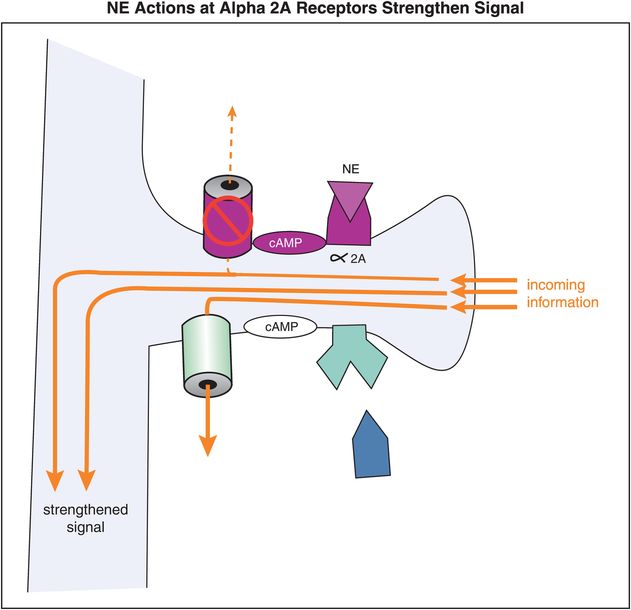
Figure 12-15. Norepinephrine actions at α2A receptors strengthen the incoming signal. Alpha2A receptors are linked to cAMP via an inhibitory G protein (Gi). When NE occupies these α2A receptors, the activated Gi-linked system inhibits cAMP and the HCN channel is closed, preventing loss of the incoming signal.
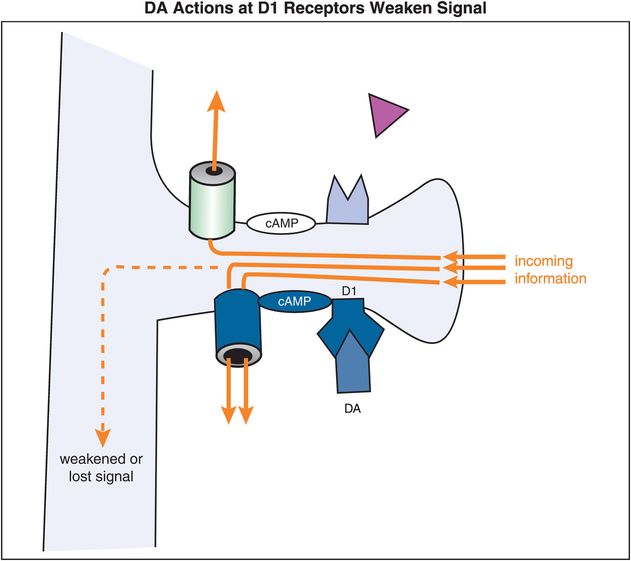
Figure 12-16. Dopamine actions at D1 receptors weaken the incoming signal. D1 receptors are linked to cAMP via a stimulatory G protein (Gs). When DA occupies these D1 receptors, the activated Gs-linked system activates cAMP, leading to opening of HCN channels. The opening of the HCN channels, especially if excessive, will lead to loss of the incoming signal before it can be passed along.
What happens following concurrent stimulation of α2A and D1 receptors by NE and DA, respectively (Figure 12-17)? While the exact localization and density of α2A and D1 receptors within various cortical areas are still under intense investigation, it is possible to imagine the same pyramidal neuron receiving NE input from the locus coeruleus (LC) on one spine and DA input from the ventral tegmental area (VTA) on another spine. If the systems are properly “tuned,” then D1 receptor stimulation can reduce the noise and α2A receptor stimulation can increase the signal to result in proper prefrontal cortex functioning (Figure 12-17). Theoretically, this will result in adequate guided attention (Figure 12-13), focus on a specific task, and adequate control of emotions and impulses.
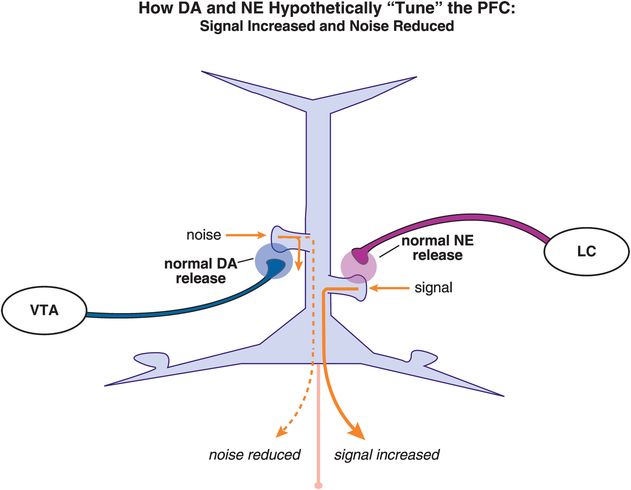
Figure 12-17. Dopamine and norepinephrine “tune” the PFC. The same pyramidal neuron may receive NE input from the locus coeruleus (LC) on one spine and DA input from the ventral tegmental area (VTA) on another spine. When properly “tuned,” D1 receptor stimulation will reduce the noise while α2A receptor stimulation will increase the signal, resulting in appropriate prefrontal cortex functioning, guided attention, focus on a specific task, and control of emotions and impulses.
What happens, however, when there is low release of both DA and NE and thus low stimulation of both D1 and α2A receptors on the spines of these pyramidal neurons (Figure 12-18)? Deficient DA and NE input will theoretically lead to increased noise and decreased signal, respectively, thus preventing a coherent signal from being sent (Figure 12-18). Hypothetically, this could cause hyperactivity, inattention, impulsivity, or some combination of symptoms, depending upon the localization of the mis-tuned pyramidal neuron in prefrontal cortex (Figures 12-3 through 12-8). Furthermore, if one neurotransmitter is low while the other is high, then a person could be exhibiting a whole different set of symptoms. By knowing both the levels of DA and NE neurotransmission and the specific area of the possible disturbances, it may one day be possible to predict the degree and type of symptoms with which a patient is ailing. With this in mind, Figures 12-7 and 12-8 show how pyramidal neurons in different brain areas may be responsible for the different symptom presentations in ADHD.
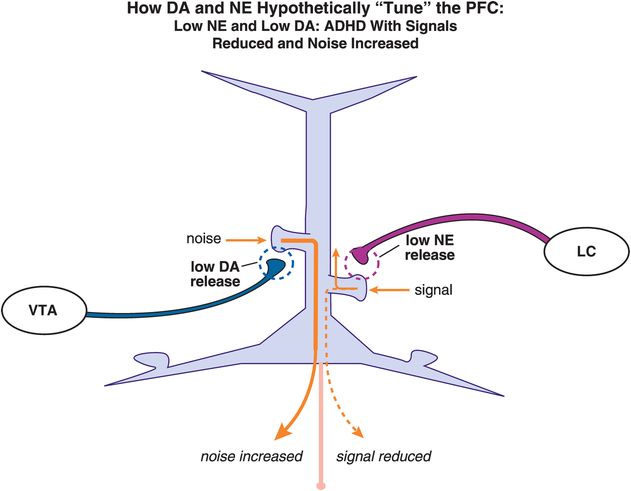
Figure 12-18. Dopamine and norepinephrine improperly “tune” the PFC in ADHD. Deficient DA will theoretically lead to increased noise, whereas deficient NE input will cause a decrease in the incoming signal. Hypothetically, this improper tuning of the PFC by DA and NE can lead to hyperactivity, or inattention, or both. Depending on the relative levels of both DA and NE, a person could display a wide range of clinical symptoms.
Neurodevelopment and ADHD
ADHD has traditionally been considered a childhood disorder, but this perspective is rapidly changing, with ADHD now being seen also as a major psychiatric disorder of adults, with some major differentiating features between ADHD in children and adolescents (Table 12-1). Nevertheless, the classic form of ADHD has onset by age seven, possibly related to abnormalities in prefrontal cortex circuits that begin before age seven but last a lifetime (Figure 12-19). Synapses rapidly increase in prefrontal cortex by age six, and then up to half of them are rapidly eliminated by adolescence (Figure 12-19). The timing of onset of ADHD suggests that the formation of synapses, and perhaps more importantly the selection of synapses for removal in prefrontal cortex during childhood, may contribute to the onset and lifelong pathophysiology of this condition (Figure 12-19). Those who are able to compensate for these prefrontal abnormalities by new synapse formation may be the ones who “grow out of their ADHD,” and this may explain why the prevalence of ADHD in adults is only half that in children and adolescents.
Children 6–12 / Adolescents 13–17 | Adults > 18 |
---|---|
7–8% prevalence | 4–5% prevalence |
Easy to diagnose | Hard to diagnose
|
Diagnosed by pediatricians, child psychiatrists, child psychologists | Diagnosed by adult psychiatrists, adult mental/medical health professionals |
High levels of identification and treatment: > 50% treated | Low levels of identification and treatment: < 20% treated |
Stimulants prescribed first- and second-line | Nonstimulants often prescribed first-line |
2/3 of stimulant use is under age 18, most of this under age 13 | 1/3 of stimulant use is age 18 or over |
1/3 of atomoxetine use is under age 18, most of this over age 12 | 2/3 of atomoxetine use is age 18 or over |
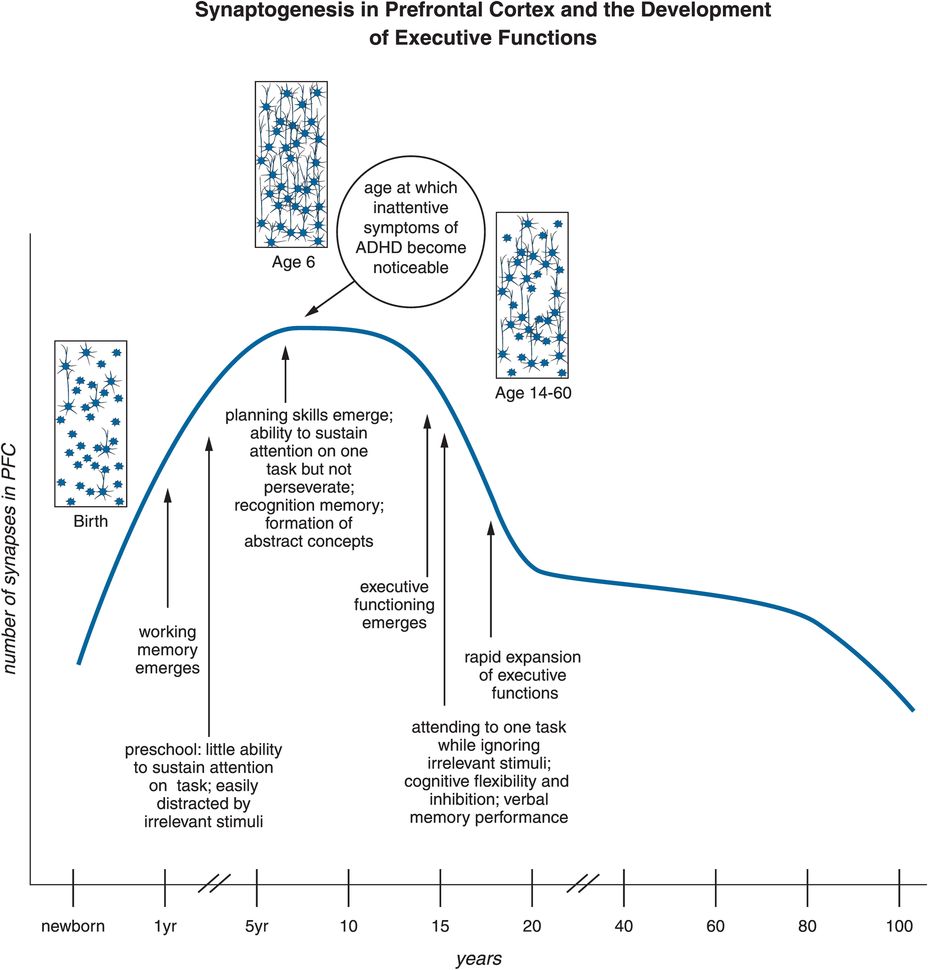
Figure 12-19. Synaptogenesis in the PFC and the development of executive functions. Synaptogenesis in the prefrontal cortex might be responsible for altered connections that could prime the brain for ADHD. Specifically, executive function develops throughout adolescence. At one year of age, working memory emerges. Around three to four years of age, children do not yet have the capability to sustain attention for long periods of time, and can be easily distracted. By age six to seven, this changes; attention can be sustained and planning can take place. This age is also characterized by “synaptic pruning,” a process during which overproduced or “weak” synapses are “weeded out,” thus allowing for the child’s cognitive intelligence to mature. Errors in this process could hypothetically affect the further development of executive function and be one of the causes of ADHD. This timeline also represents when symptoms of ADHD often become noticeable, which is around the age of six.
What causes these problems in the circuits of the prefrontal cortex in ADHD? Currently, leading hypotheses propose that neurodevelopmental abnormalities occur in the circuits of the prefrontal cortex in ADHD (Figures 12-2 through 12-8). In fact, genes that code for subtle molecular abnormalities are thought to be just as important to the etiology of ADHD as they are to the etiology of schizophrenia. Many of the ideas about the neurodevelopmental basis of schizophrenia, such as abnormal synapse formation and abnormal synaptic neurotransmission, serve as a conceptual framework and neurobiological model for ADHD as well. The genetic factors linked to schizophrenia are discussed extensively in Chapter 4. The major genes implicated in ADHD are those linked to the neurotransmitter dopamine, although links to the genes for the α2A-adrenergic receptor, serotonin receptors, and some other proteins are also under intense investigation. Environmental factors inevitably contribute to ADHD, as they do to so many other psychiatric disorders. This includes factors such as preterm birth, maternal smoking during pregnancy, and others.
The impact of neurodevelopment on the specific symptom patterns of ADHD is shown in Figure 12-20. Inattentive symptoms are not really seen in preschool children with ADHD, perhaps because they do not have a sufficiently mature prefrontal cortex to manifest this symptom in a manner that is abnormal compared to normal development. Preschool ADHD and its treatment are a current controversial concept in the field, because most studies of stimulants involve children over the age of six. Once inattention becomes a prominent symptom of ADHD, it remains so over the life cycle (Figure 12-20). However, hyperactivity declines notably by adolescence and early adulthood, while recognized comorbidities skyrocket in frequency as ADHD patients enter adulthood (Figure 12-20).
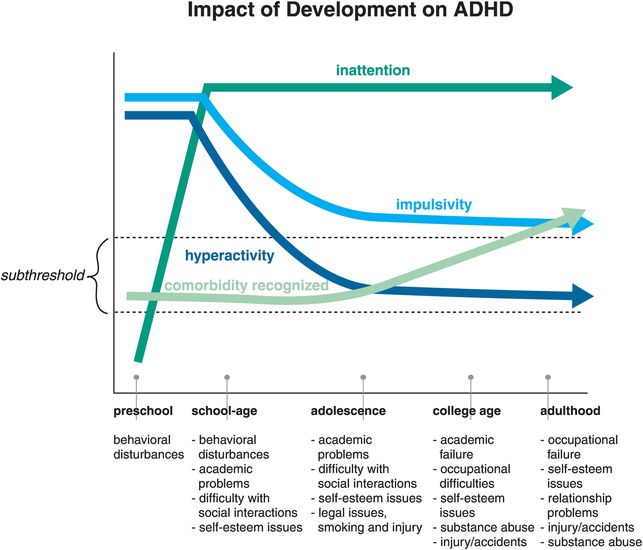
Figure 12-20. Impact of development on ADHD. The evolution of symptoms across the ages shows that although hyperactivity and impulsivity are key symptoms in childhood, inattention becomes prevalent as the patient ages. Additionally, the rates of recognized comorbidities increase over time. This could be due to the fact that the comorbidities were overlooked in children with ADHD, or because ADHD was never diagnosed in some patients presenting with anxiety or learning disabilities. One could say that “the jury is still out” on this issue.
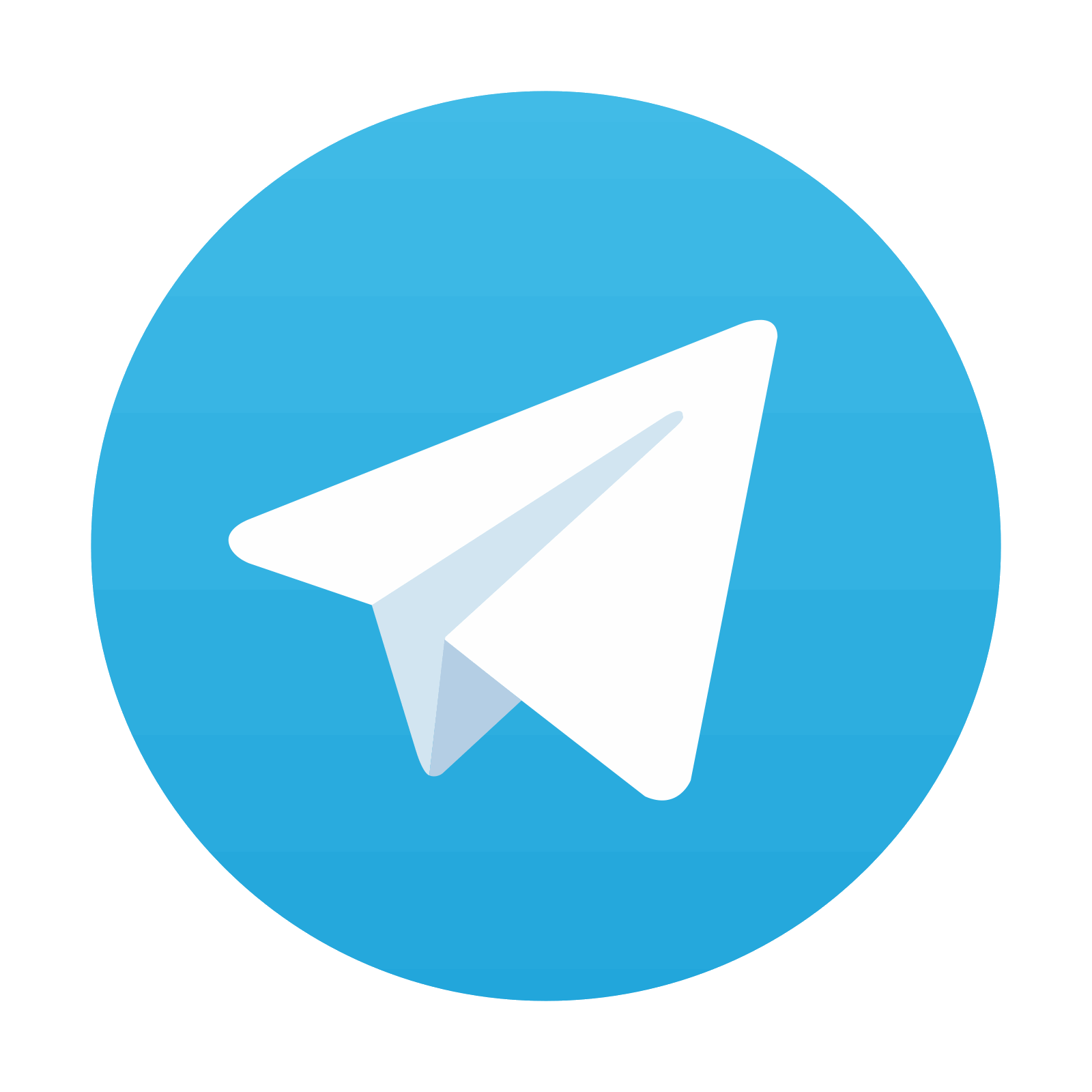
Stay updated, free articles. Join our Telegram channel
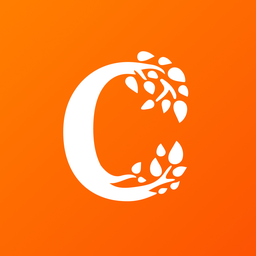
Full access? Get Clinical Tree
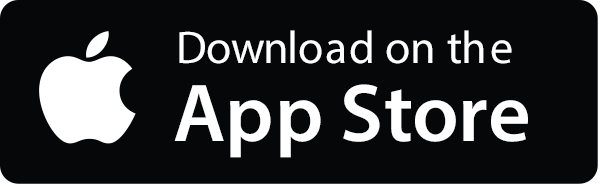
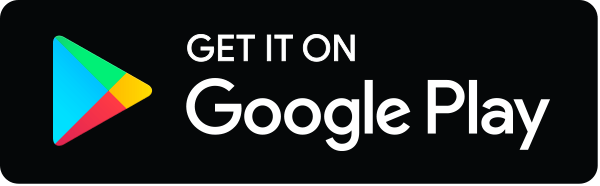