Polyacrylamide Gel Electrophoresis (PAGE) [Sodium Dodecyl Sulfate (SDS)/Non-SDS]
PAGE is a laboratory technique designed to separate proteins according to their size, shape, and also charge. Proteins for PAGE are usually prepared by placing them in an anionic (negative charge) detergent mixture, which, along with heating to approximately 60°C, denatures the protein, breaks any cysteine–cysteine disulfide bonds, and creates a fairly linear protein structure. PAGE can also be run with an addition of SDS. SDS, if used, is included in the detergent mixture and assists in disrupting the proteins’ secondary and tertiary structures. In addition, SDS binds to each peptide chain in a ratio of one SDS per two amino acid residues, thereby adding a negative charge proportional to the peptide length. The addition of SDS to PAGE samples, therefore, also makes the proteins’ shapes and native charges irrelevant; only the total length and the resulting SDS charge matters.
Polyacrylamide gel is composed of linear acrylamide molecules, cross-linked by bisacrylamide via the catalytic actions of ammonium persulfate and tetramethylethylenediamine (TMED). Cross-linking forms a web-like polyacrylamide lattice with pores of approximately the same size throughout. Varying the amounts of acrylamide, bisacrylamide, and aqueous solution allows scientists to accurately vary the pore size and, therefore, the relative ability to separate differently sized protein molecules.
After polymerization, the gel is placed in an electrophoresis device and immersed in a buffer with a cathode (positive charge) at the top and an anode (negative charge) at the bottom. The protein samples are “loaded” onto individual gel lanes formed by a removable comb. A tracking dye is usually included in the samples to monitor the progress of the proteins through the gel.
The electric field causes the negatively charged proteins (charge usually enhanced by the proportional negative charge of SDS molecules) to move toward the anode proportional to their length (and, therefore, charge). Movement through the gel is not directly proportional to the overall charge, though, because the larger proteins will encounter much more difficulty in moving through the pores because of their size and linear rigidity. Although the length of each peptide increases the electrical force moving it down the gel, the “filtering” action of the gel pores will allow smaller molecules to travel further down the gel, whereas larger molecules remain near the top.
(A) The PAGE system employs the electric charge deployed between a positive electrode (anode) and negative electrode (cathode). (B) Proteins are loaded (origin) onto polyacrylamide gel, which is exposed to this electric field. Negatively charged proteins are driven toward the anode, whereas positively charged proteins will remain nearer to the cathode. The relative movement of each protein depends on its specific charge determined by its amino acid composition. When SDS is not used (“native PAGE”), the secondary-to-quaternary structure of each protein also influences the movement through the acrylamide gel matrix. When SDS is used, higher order structure is eliminated, separation by charge predominates. [Reproduced with permission from Naik P: Biochemistry, 3rd edition, Jaypee Brothers Medical Publishers (P) Ltd., 2009.]
When the tracking dye approaches the bottom of the gel, the electrical field is stopped and the gel is stained using a variety of chemicals or solutions, which bind to proteins (e.g., Coomassie blue, silver) to allow visualization in the gel. Proteins of known molecular weight, referred to as “molecular markers,” are normally run in a separate lane to allow direct comparison with the proteins from the experimental sample. PAGE gels can be used further for western blotting (see below) and other biochemical techniques.
SDS or non-SDS PAGE is often used during the biochemical isolation of a single protein from tissue or protein mixtures, although it can also be used clinically for diagnostic and/or treatment purposes. Because of its ability to dissolve molecules, SDS is sometimes used in enemas as a laxative.
Markers of known molecule weight (kDa) are loaded in one gel lane (left) to allow characterization of proteins separated on the other lanes of the gel. [Reproduced with permission from Murray RA, et al.: Harper’s Illustrated Biochemistry, 28th edition, McGraw-Hill, 2009.]
Two-dimensional (2D) PAGE is performed in two directions on the same sample, offering improved separation for complex mixtures of molecules. In this technique, samples are exposed to an electric current for a first separation. The gel is then turned 90° and a current is again applied with an alternative buffer, which affects the movement of the samples differently. As a result, a second separation is achieved. Examples include molecular weight separation followed by separation based on the molecules’ overall pH, known as the “isoelectric point.” 2D electrophoresis is used in many clinical conditions as a preparatory step before western blotting (see below) for tests such as confirmation of human immunodeficiency virus (HIV) and hepatitis B infection, the detection of prions in Creutzfeldt–Jakob disease, also known as “mad cow disease,” and diagnosis of Lyme disease, among others.
Samples are run via normal SDS–PAGE (shown in vertical direction), which separates the proteins based on size, turned 90°, and run a second time via isoelectric focusing (shown in horizontal direction) technique with a pH gradient, which separates the proteins based on total pH of the protein (see text). Running the same sample by these two different techniques allows increased separation of complex mixtures of proteins and/or separation of proteins of the same size, but differing isoelectric points. [Reproduced with permission from Murray RA, et al.: Harper’s Illustrated Biochemistry, 28th edition, McGraw-Hill, 2009.]
Immunoassays
Immunoassay is a generic term for the application of antibodies to biochemical testing. These techniques paved the way for a wide variety of techniques of separation, measurement, and positive identification of biological molecules, and include radioimmunoassays (RIA), enzyme-linked immunosorbant assay (ELISA or EIA), and western blotting.
All immunoassays involve the addition of an antibody specific for a known molecule (antigen) to a solution presumably containing, at least in part, that molecule. If the antigen is present, the antibody will bind specifically and, if properly chosen and prepared, strongly. Monoclonal antibodies will normally bind the tightest and with the greatest specificity; polyclonal antibodies are usually weaker and less specific in their binding patterns. Selective precipitation or chromatography (see below) can be used to isolate and purify the antibody–antigen complex as needed.
If a chemical or another label has been previously attached to the antibody, it can be used to detect the presence and, in many cases, the quantity of the antigen. Examples of these detection techniques include fluorescent molecules, enzymes that produce a particular color when provided an appropriate substrate, radioactive or magnetic labels, and even gold particles, which coalesce to form a visual precipitate. Alternatively, another antibody with a radioactive detector molecule, which selectively binds to the first antibody, can be added later in the test. Immunoassays are used in a multitude of clinical tests, especially for the detection of infections in blood, urine, cerebral spinal fluid, and so on.
The first immunoassay developed was the RIA. In this test, a molecule of interest, such as a hormone, has a radioactive molecule (e.g., iodine-125, carbon-14, or hydrogen-3) attached to it. The researcher also has an antibody (monoclonal or polyclonal), which specifically recognizes and binds to this hormone.
Detection by RIA
A molecule of interest (A) can be detected by placing a radioactive label (yellow starburst) onto a protein (B), which is known to bind to (A). Important examples of protein (B) include hormones and monocolonal and polyclonal antibodies. [Adapted with permission from Mescher AL: Junqueira’s Basic Histology Text and Atlas, 12th edition, McGraw-Hill, 2010.]
A measured amount of the antibody and a measured amount of the pure hormone (both radiolabeled and unlabeled forms) are added to assay tubes with concentration of the unlabeled hormone greatly exceeding that of the radiolabeled hormone. Because only a small amount of antibody is added and its capacity to bind the hormone is limited, the antibody-binding sites will be saturated (i.e., all occupied; see figure below) As a result, only a fraction of the total amount of hormone will be actually bound to the antibody. Given that the antibody cannot differentiate between labeled and unlabeled hormones, both forms of the hormone will compete for the limited number of binding sites on the antibody. When a small amount of unlabeled hormone (i.e., from standard or patient sample) is added to the assay, then only a small amount of the bound-labeled hormone is displaced from the antibody. As the amount of unlabeled hormone increases, the amount of radiolabeled hormone bound to the antibody decreases considerably.
A variety of techniques can be used to separate unbound hormone from the antibody-bound hormone. Therefore, direct measurement of bound-labeled hormone can be easily made. Using known quantities of unlabeled hormone, it is possible to construct a standard curve which compares the amount of radiolabeled hormone bound to the antibody with the amount of pure unlabeled hormone added to the tube. By replacing pure hormone with a patient sample, the concentration of the hormone in the sample can then be directly determined.
Standard Curve of Hormone Binding
A specific antibody to the hormone is incubated with the radiolabeled hormone. A standard curve is created by introducing known concentrations of unlabeled hormone to displace the radiolabeled hormone. The amount of radioactivity remaining is a function of the unlabeled hormone concentration. When unknown samples are used, the measurement of the radioactivity remaining allows the hormone concentration to be determined from the standard curve. [Adapted with permission from Kibble JD and Halsey CR: The Big Picture: Medical Physiology, 1st edition, McGraw-Hill, 2009.]
One drawback of this method is that RIA measures the immunologic activity, not the biological activity, of a hormone. Ideally, both a bioassay and RIA are evaluated. However, in a clinical situation, with a few rare exceptions, using the RIA provides sufficiently accurate information on hormone levels in the patient sample. The advantages of RIA are sensitivity and specificity as well as rapid and economic evaluation of patient blood or urine samples.
The principle of RIA is the basis of newer methods of measurement, for example, ELISA or EIA. The ELISA technique uses two different antibodies with varying binding affinity to a molecule that the researcher wishes to detect or study. An example is a polyclonal or a monoclonal antibody made against the same molecule. Normally, a microtiter plate, containing multiple test wells coated with the polyclonal antibody (not shown in figure), is used. The test samples are placed in the wells and the antigen, if present, is allowed to bind to the antibody. After a suitable time period, the remainder of the test sample is removed and the wells are washed to remove nonspecifically bound molecules. Next, a monoclonal antibody against the same antigen is added to the wells and allowed to bind to the antigen molecule. The use of polyclonal and monoclonal antibodies usually allows multiple binding to the same antigen molecule. Detection techniques are then used to determine the presence and quantity of the antigen being tested. One common method is to use an enzyme attached to the antibody, which produces a colored product. After the binding of the second antibody, addition of the enzyme’s substrate creates a color reaction, which can often be quantitated (see the figure below). Detection using radioactive or fluorescent molecules attached to the second antibody can also be utilized. ELISA is used for a wide variety of clinical tests (e.g., identification of HIV, allergens, and/or drugs), especially if the concentration of a certain protein or other antigen must be determined. The ELISA technique is also the basis for home pregnancy tests, where the hormone binds first to an antibody–enzyme complex with detection and quantization via a second antibody containing a dye that is produced by an enzyme.
Overview of ELISA Testing Methods (Direct and Indirect)
Antibody to an antigen being tested is applied to the sample. Following binding, the antigen can be detected either via an attached enzyme on the primary antibody (direct method, left) or by the addition of a secondary antibody with an attached enzyme (indirect method, right). When substrate is added to the antibody–antigen sample, the enzyme produces either a fluorescent or colored product, allowing easy measurement, which allows both quantitative and qualitative assessment of the original antigen. [Adapted with permission from Mescher AL: Junqueira’s Basic Histology Text and Atlas, 12th edition, McGraw-Hill, 2010.]
Chromatography
TLC or paper chromatography relies on a thin layer of material (e.g., paper, cellulose, silica, and others) with varying absorbance for molecules. Although often used for nonbiological molecules, TLC can be utilized for protein and lipid separation and/or identification. A small volume of each sample for analysis is placed in vertical lanes close to one end of the TLC layer, and that end is partially immersed in a solvent, ensuring that the sample is not covered by the solvent. This solvent is drawn through and up the TLC material by capillary action. As it travels, it carries the sample molecules up with it at rates depending on the molecule and solvent properties, including the level of adsorption onto the chromatography layer and the solubility of the sample in the liquid (see the following figure). As a result of the varying solid and liquid layer affinities, separation of a mixture of molecules results. The TLC layer is then “developed” for molecule detection via chemical or spectroscopic means. In the case of a single, purified molecule, the distance traveled on a specific TLC with a specific solvent can provide identification by comparison with known standards. Two-way TLC can also be used, in which after separation by one solvent, the sheet is turned 90° and exposed to the actions of a second type of solvent. Two-way TLC offers improved separation for some molecules.
TLC
(A) Diagram of TLC equipment, illustrating TLC support medium, solvent (light brown), point of application of three samples (black dots), and direction of movement (indicated by arrow) of molecular samples as the solvent moves up the medium. (B) Actual TLC plate showing known sample of G (left lane) and L (middle lane) and separation of a mixture of these two proteins into the component parts (right lane). [Adapted with permission from Naik P: Biochemistry, 3rd edition, Jaypee Brothers Medical Publishers (P) Ltd., 2009.]
Column chromatography utilizes the same principles of TLC, but in a three-dimensional column system that allows much larger amounts of sample to be separated and studied. A glass tube or “column” is filled with a selected material, called stationary phase, which has particular chemical qualities (e.g., hydrophobic, hydrophilic, anionic (–) charges, cationic (+) charges, etc.). Molecules will bind to the stationary phase, depending on their individual chemical qualities. For example, a protein with a strong hydrophilic external character will not want to bind to a hydrophobic column material, but will want to bind to a hydrophilic one. The variation in binding of each molecule leads to separation because molecules that bind less will travel more quickly through the column and vice versa. Often, the molecules will bind to the stationary phase until another liquid solvent, called mobile phase or eluent, with stronger hydrophobic/hydrophilic or anionic/cationic qualities is run through the column. Sometimes, sequential eluents, each with a different chemical quality, are run through the column, each causing selected molecules to unbind and flow out of the column. The material that passes through the column is collected in small samples of a known volume, called fractions, for further study. Often, molecules in the fractions are monitored by light [e.g., ultraviolet (UV)] absorption or other detection methods.
Gel filtration chromatography, a variation of column chromatography, is used for the biochemical separation of biological molecules, mainly proteins and nucleic acid strands, depending on the size and shape of the molecule. The technique usually preserves biological function. Gel filtration uses beads formed from polyacrylamide (see above), dextrans, or agarose (see Chapter 2) with pores of approximately equal size. The particular pore size of each type of gel filtration bead depends on their exact materials and different fabrication techniques whose characteristics allow researchers to optimize column chromatography for varying samples.
Biological samples are applied to the top of the column in a small volume. A solution is run through the column carrying the sample through the gel beads. Small and/or predominately compact globular molecules are able to enter the bead pores and, therefore, have a large volume available to them. This large volume results in a slower transit time through the column. Larger particles or ones with a noncompact or linear structure are unable to enter the bead pores and travel more quickly through the smaller volume between the beads. The molecular size and shape of each biological molecule applied to the column directly determine how fast or slow it travels through and out (elution) of the column (see figure below). This varying speed results in separation.
Gel Filtration Chromatography
Small molecules (blue) are able to enter the gel beads, increasing their available volume and slowing down the speed of travel through the column. Larger molecules (red) are unable to enter the gel beads and travel more quickly through and out of the column. As a result, molecules separate and elute from the column at a rate proportional to their molecular weight/size. [Reproduced with permission from Naik P: Biochemistry, 3rd edition, Jaypee Brothers Medical Publishers (P) Ltd., 2009.]
Ion-exchange chromatography, another type of column chromatography, takes advantage of charged groups (e.g., ions) that are part of the gel bead material. Different biological molecules interact more or less with these chemical groups (e.g., a more negative molecule will be attracted to positively charged gel beads), altering their speed of elution and resulting in separation. Some molecules will bind so strongly to the charged gel beads that a solution with a stronger ionic charge must be used to displace or “elute” them.
Ion-Exchange Column
Gel beads with a positive ionic charge attract negatively charged molecules (blue) more than positively charged ones (orange). This different affinity, based on charge, allows separation of various molecules. [Reproduced with permission from Naik P: Biochemistry, 3rd edition, Jaypee Brothers Medical Publishers (P) Ltd., 2009.]
Affinity chromatography works much like ion-exchange chromatography (above), but takes advantage of specific molecules that are part of or previously attached to the gel bead material. As an example, antibodies coupled to the gel material are sometimes used for very specific binding to the desired molecule. Other examples of affinity chromatography include the use of gel-bound substrates or coenzymes. The biological molecules remain bound to the gel bead until a second solution of changed ionic strength or pH is applied to affect their release and elution.
Regardless of the particular type of column chromatography used, eluted biological molecules are collected in small, ordered volume allotments, known as fractions, and the molecules are detected by spectroscopic or chemical identification methods.
HPLC is a technique used for enhanced and precise separation of even small amounts of biological and nonbiological molecules. HPLC columns utilize variations of the separation principle behind column chromatography, depending on variable attractive and repulsive forces on the gel material and their interaction with the biological molecules being studied. HPLC also utilizes smaller columns than regular column chromatography, with more specialized gel materials. The smaller columns and resulting tighter packing of the gel material in HPLC combined with the use of a pump to propel the column solution (sometimes organic solvents) either in a single solution or as a gradient of different solutions under high pressure lead to fast, efficient, and more selective separation of molecules that may not be possible with other techniques. Methods to detect these molecules once they are eluted, including light absorbance, fluorescence, electrochemical, and others, are similar to those utilized in column chromatography.
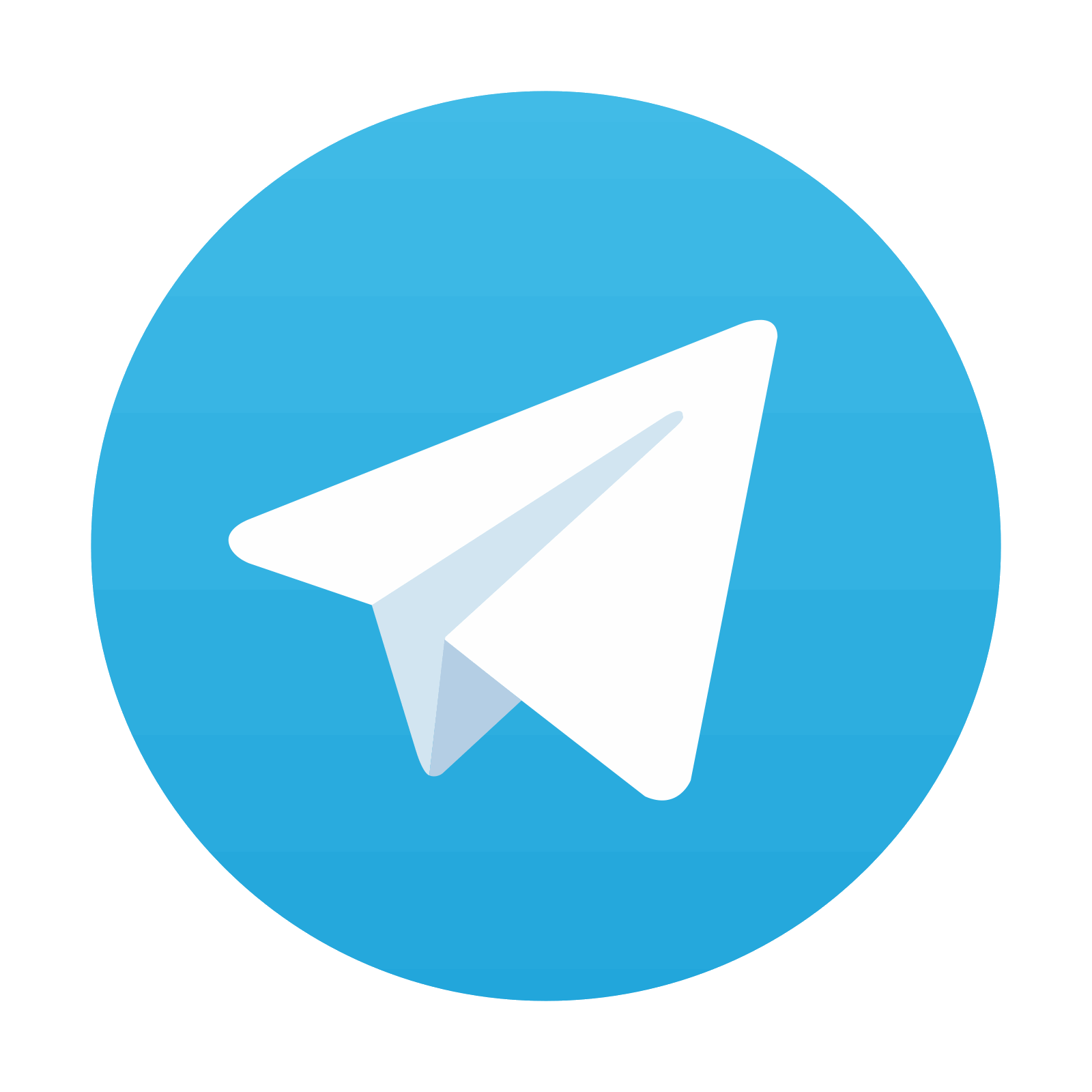
Stay updated, free articles. Join our Telegram channel
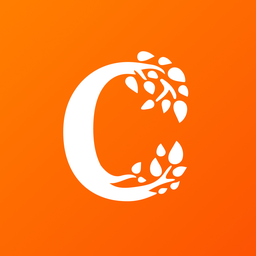
Full access? Get Clinical Tree
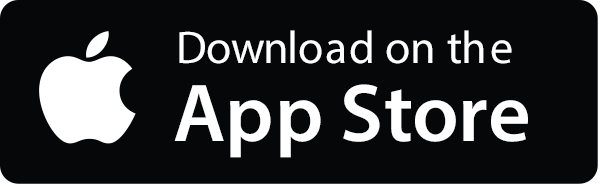
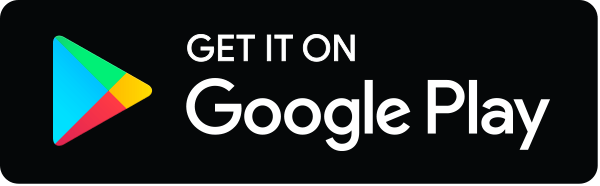