Fig. 4.1
A highly stylized auditory hair cell is drawn in this schematic. The cell is depicted to be under the stress of typical pathological triggers of noise, aging, and ototoxicants. Noise induces increased generation of free radicals via reduced cochlear blood flow, ischemia–reperfusion mechanisms, and increased metabolism also leading to increased free radical production by the mitochondrial electron transport chain. Aging, by reducing the effectiveness of antioxidant defenses (such as glutathione [GSH], glutathione peroxidase [GSHPx], glutathione S-transferase [GST], superoxide dismutase [SOD], and catalase), exacerbates oxidative stress. Ototoxicants like the aminoglycoside antibiotics or the cytotoxic compound, Cisplatin, act to interfere with antioxidant defenses, bind RNA and DNA, and activate iNOS, thereby stimulating the production of NO. ROS, in turn, damage the cell’s plasma membrane as well as the membranes of organelles like the mitochondrion, including its genome. This outcome initiates the release of cytochrome c (Cyt c) and the caspase cascade leading to DNA fragmentation and apoptosis
4.4.1 Early Evidence
Historically, Hawkins and his colleagues were the first to note the parallels between cochlear damage observed in noise-induced injury and the type of otopathology seen in aminoglycoside toxicity (Hawkins 1973). They also reported that there was an apparent progression of the severity of injury progressing from cochlear base to its apex and from the OHC rows inward from the periphery. Also in the early part of the decade of the 1970s, Ylikoski reported swelling and distortion of the OHCs in guinea pigs exposed to aminoglycosides (Ylikoski et al. 1974). In addition, these investigators also reported significant subcellular distortions and damage in the OHCs. A few years later, Fredelius et al. examined the consequences on the cochlea of intense continuous noise exposure and reported that the stereociliary bundle was disrupted in IHCs and OHCs along with cuticular plate protrusions (Fredelius et al. 1988). In addition, these investigators described how the afferent nerve endings below the IHCs were swollen and translucent and that intracellular organelles (including mitochondria) were seen to degenerate. It is now clear that oxidative stress contributes to all of the above patterns of damage, and multiple recent reviews are available (Kidd and Linden 1975; Rybak and Whitworth 2005; Abi-Hachem et al. 2010; Poirrier et al. 2010).
4.4.2 Unique Vulnerability of the Cochlea to Oxidative Injury
As noted, the cochlea and organ of Corti are a highly energetic system. In order for the cochlea to transduce sound impulses, the stria vascularis is compelled to maintain an electrochemical gradient between the hair cells and the endolymph (Kopke et al. 1999). In addition, the OHC tuning of the basilar membrane is an energy-dependent process (Patuzzi 1996). Accordingly, the stria and the OHCs are mitochondrion-rich structures. Under physiologic conditions, the reactive species that are generated by the metabolic activity in these regions are effectively scavenged by endogenous mechanisms or oxidant neutralization (Thalmann et al. 1973). It is now understood that when conditions change for the worse, such as under the stress of intense noise exposure or the presence of ototoxins, there exists the potential that theses defenses will be overwhelmed resulting in oxidant-induced injury.
4.4.3 Noise and Reactive Species
It has been shown that noise exposure increases the rate of metabolism in the cochlea. If homeostatic systems are undisturbed or uncompromised, ordinary levels of noise exposure and its molecular effects are readily contained by the cochlear antioxidant defenses. Early studies demonstrated that antioxidant compounds and ROS scavengers could protect against noise-induced hearing loss (NIHL) (Quirk et al. 1994). However, overstimulation by intense sound can easily perturb the protective systems leading to increased glucose uptake and increased perilymphatic oxygenation (Kopke et al. 1999). With further increases in incident noise, decreased CBF and declining endolymph oxygenation and glucose uptake are subsequently noted (Wangemann and Schacht 1996). This is fertile territory for a robust generation of ROS. Interestingly, NO has also been shown to operate as a neuromodulator in the central nervous system but can prove to be, in conducive settings, neurotoxicity, and indeed if enough of this short-lived molecule is generated (as outlined briefly above), it can act as an oxidant itself (Fessenden et al. 1994). In addition, calcium which is a tightly regulated messenger in the cochlea (as in many other tissues) can itself trigger ROS production when intracellular concentrations ([Ca2+]i) increase (Orrenius et al. 1992). The effects of noise on the inner ear are reviewed in detail by Altschuler in Chap. 7, and the genetics of NIHL are reviewed by Yamashita in Chap. 8.
4.4.4 Oxidant Stress and Cochlear Apoptosis
A large body of evidence suggests that oxidative stress in the cochlea, regardless of origin or trigger, will initiate apoptotic cascades in the hair cells as well as in the neurons of the spiral ganglion (Huang et al. 2000). As described, ROS-induced stress provokes the mounting of cellular defenses in the cochlea. Scavengers that are utilized, normally with good effect by the hair cells and neurons, include SOD, catalase, glutathione peroxidase (GPx), and glutathione (GSH). GSH, for example, acts to attenuate the oxidation of proteins by ROS. It is also known to function as a detoxifier of 4-hydroxynonenal (HNE, a plasma membrane lipid peroxidation by-product that is very toxic). If HNE detoxification is reduced, increasing levels of this molecule in the cell can injure the mitochondrial membrane resulting in cytochrome c (Cyt C) release as has been described by classical experiments in recent years (Ji et al. 2001; Vieira et al. 2001; Rybak and Whitworth 2005) . Release of Cyt C is a well-understood trigger for apoptotic signaling to be initiated (Kruman et al. 1997). In other models of cochlear injury such as neurotrophin withdrawal, cisplatin exposure, hypoxia, or ischemia as well as noise exposure, apoptotic cell death has been observed in the cochlea. In general, in such models, treatment with a cell permeant type of glutathione monoethyl ester (GSHe) was shown to be protective, further bolstering the notion that apoptosis is an important outcome in cochlear injury from ROS (Kopke et al. 1997; Ohinata et al. 2000).
4.4.5 Oxidant Stress in Aminoglycoside Toxicity
Aminoglycosides are a large family of microbial protein synthesis inhibitors that have seen wide use as antibiotics. They include drugs such as amikacin, arbekacin, gentamicin, kanamycin, and neomycin. Of these, gentamicin and kanamycin have been particularly implicated in enhancing the generation of hydroxyl radicals in cochlear explants (Clerici et al. 1996). Published reports have shown that these compounds likely produce ROS by acting as iron chelators (Song and Schacht 1996; Priuska and Schacht 1997). This is underscored by the demonstration by several investigators that both iron chelators and ROS scavengers have proven to be effective moderators of aminoglycoside-induced injury (Song and Schacht 1996; Priuska and Schacht 1997). A contrasting argument (e.g., by Basile et al.) has implicated overstimulation by N-methyl-d-aspartate (NMDA) by aminoglycosides as the basic mechanism of toxicity in the cochlea (Basile et al. 1996). These authors were able to attenuate hearing loss and hair cell damage by the use of NMDA antagonists demonstrating that aminoglycoside toxicity is excitotoxic in part. Indeed, glutamate release is also a trigger for ROS generation which fits with this alternate picture of NMDA overstimulation (Khan et al. 2000). The role of oxidative stress in aminoglycoside ototoxicity and the use of antioxidants as therapeutics are described in detail in Chap. 10 by Rybak.
4.4.6 Cisplatin Ototoxicity and ROS
Cisplatin [cisplatinum, cis-diamminedichloridoplatinum (CDDP)] is a commonly used cytotoxic drug used in cancer chemotherapy. It acts by cross-linking DNA and triggering apoptosis, preferentially in malignant cells. The drug remains useful despite its known toxicity toward the kidney, nervous system, and cochlea (Ravi et al. 1995). Cochlear toxicity manifests as tinnitus followed by hearing loss above 4 kHz (Rybak 1981). Such ototoxicity is often bilateral and tends to correlate with the frequency of cisplatin administration (Powis and Hacker 1991).
The evidence for the involvement of ROS includes observations in cochlear explants of free radicals by electron paramagnetic spin spectroscopy following cisplatin exposure (Clerici et al. 1996). Further, ROS-induced cochlear damage was noted to be accompanied by an accumulation of malondialdehyde, a well-established marker of ROS-related cellular damage (ensuing from the oxidation of polyunsaturated fatty acids), a key marker of ROS-induced membrane damage, as well as a decrease in GSH following exposure to cisplatin and decreased GPx and reductase activity with an increase in SOD and catalase activity in the inner ear (Ravi et al. 1995; Kopke et al. 1999). In addition, ROS have also been detected inside cisplatin-exposed hair cells using vital dyes and confocal microscopic imaging (Ravi et al. 1995; Kopke et al. 1999). Finally, in certain experiments, when the cochlea was observed in the presence of buthionine sulfoximine (a potent GSH synthesis inhibitor), auditory hair cell loss was accentuated (Kopke et al. 1999). The role of oxidative stress in cisplatin ototoxicity and the use of antioxidants as therapeutics are described in detail in Chap. 11 by Laurell.
4.4.7 ROS in Cochlear Senescence
Age-related hearing loss (ARHL; presbycusis) is the most common hearing deficit in those of advanced age and has become a significant social and public health issue (Huang and Tang 2010). It appears that a lifetime of exposure to noise, genetic susceptibility, a variety of otologic and systemic disorders, and toxic agents are the main etiologic factors (Gates and Mills 2005). In early phases of presbycusis, a high-frequency hearing loss is seen, and the trouble with consonants such as P, H, G, CH, SH, K, F, S, and TH can begin even with mild hearing later. Later, the individual begins to have difficulty in detecting, identifying, and localizing sound sources. Once hearing loss progresses to the 2–4 kHz frequency range, with vowel understanding also compromised, speech comprehension is further compromised.
4.4.7.1 Free Radicals and Damage to Mitochondrial DNA with Age
In the 1950s, Harman proposed a free radical theory of aging based on evidence that was beginning to implicate ROS-induced subcellular damage, defects, and dysfunction (Harman 1956). Subsequent to this novel and intriguing idea being proposed, Miquel and colleagues advanced the mitochondrial degeneration mechanism of senescence (Miquel et al. 1980, 1992; Miquel and Fleming 1984; Miquel 1991). In summary, Miquel’s view is that ROS cumulatively injure the circular mitochondrial genome, and because this is the critical repository of information for the bioenergetics machinery, ATP synthesis and regulation becomes compromised over time (Huang and Tang 2010). Miquel’s group was one of the early reporters of the phenomenon of life span extension in laboratory animals whose diet was supplemented with antioxidants (Miquel 2002). Disappointingly, this finding has not extended to nonempirical, real-life settings, unless evidence is limit to Drosophila (Peng et al. 2014). However, the underlying mechanisms appear to be valid and reproducible within rodent models, and important studies have shown that murine life spans are measurably lengthened when ROS defenses are bolstered. An excellent example of such a report is from Schriner and his colleagues who found life span increases in mice overexpressing catalase targeted to the mitochondria (Schriner et al. 2005). Equally elegant is that older experiments found that antioxidants had little or no impact on murine life spans. Interestingly, in the classic paper by Kohn, antioxidants were able to eke out an effect if controls were exhibiting compromised survival relative to mean population data (Kohn 1971). It is obvious that this is the primary caveat in these studies since it is unclear if the findings are extendable up the phylogenetic scale.
Specifically, evidence has been found that in aged C57Bl6/J mice, antioxidant mRNA levels decline progressively (Staecker et al. 2001). In addition, McFadden et al. have observed that SOD1 and O2− are also implicated in the pathogenesis of ARHL which can be attenuated by therapeutic strategies directed at these molecules (Staecker et al. 2001). Jiang has found measurable levels of ROS in the senescent cochlea (Jiang et al. 2007). In old rats, investigators have also seen evidence that GSH levels are significantly reduced in the eighth nerve (Lautermann et al. 1997). Researchers have strengthened the mitochondrial DNA damage idea in successfully testing for mutations in the mitochondrial DNA and reporting that these are positive determinants of hearing loss in elderly human subjects (Fischel-Ghodsian et al. 1997). Seidman and colleagues demonstrated that a 4,834-bp mitochondrial DNA deletion (mtDNA) was present in older subjects with ARHL and went on to identify a 4,977-bp mtDNA defect in archived human temporal bone specimens from patients with ARHL (Bai et al. 1997; Seidman et al. 1997, 2004). Additional mtDNA deficits have also been identified which correlate with ARHL. Examples are the 5,142-bp and 5,354-bp deletions in mtDNA reported by Markaryan et al. (2008). Finally, certain mtDNA haplogroups have been shown to be associated with ARHL (Manwaring et al. 2007). Thus, ample evidence not only implicates ROS in being responsible for subcellular defects and dysfunction in the aging organism but, more specifically, the bioenergetics nexus of the cell, the mitochondrion, appears to be the site where these reactive species, both in the short-term and in a chronic sense, create anomalies that lead to perturbations and loss of function.
4.5 Antioxidants as Therapeutic Agents
When looked upon as a typical biological system, ROS and their associated cellular signaling mechanisms manifest complex and parallel architecture such as is seen in most cellular signaling networks. This picture is compounded by the fact that ROS include many different species with varying dynamics and properties. Thus, it is not surprising that most medical interventions that target ROS have not been successful (Steinhubl 2008). Contributing to this observation is the notion that ROS-based signaling is spread across a relatively narrow range from adaptive to maladaptive (Nathan and Cunningham-Bussel 2013). The disappointment of unsuccessful ROS targeting in human disease as well as in many animal models of human pathology is somewhat balanced by the fact that many successful drugs work by impacting ROS signaling or by sensitizing cells to such signals as well as diminishing ROS generation (Nathan et al. 1981; Liou and Storz 2010; Raj et al. 2011). In the ear, the status of science is similar and we will examine it in some detail below.
4.6 NIHL and Antioxidants
It has to be stated at the outset that there is no FDA-approved drug that is currently available to prevent or treat NIHL (Lynch and Kil 2005). However, many studies in animal models of NIHL have reported robust attenuation of NIHL by agents that diminish or protect from the effects of reactive species (Henderson et al. 1999, 2006; Le Prell et al. 2003; Lynch and Kil 2005). One of the early entrants in antioxidant research for the treatment or prevention of NIHL was the compound allopurinol, a xanthine oxidase inhibitor used to treat hyperuricemia. With systemic injection of allopurinol during and after noise exposure, allopurinol reduced the resulting permanent hearing loss (Seidman et al. 1993). Later data suggested this effect was limited as allopurinol was only able to rescue NIHL if the threshold shift was temporary (TTS) and not when the noise exposure was high enough or long enough to cause a permanent shift in threshold (PTS) as assessed 15–30 days post noise exposure (Franze et al. 2003).
More recently several other antioxidants have been used with interesting results. These include the GSH precursors N-acetylcysteine (NAC) and methionine (MET). When injected intraperitoneally, both NAC and MET were shown to be otoprotective (Kopke 2001). It is worth to note there are at least two studies with human subjects in which TTS was reduced by an antioxidant agent, including alpha-lipoic acid (Quaranta et al. 2012) and vitamin B12 (Quaranta et al. 2012). In 2011, Lindblad and colleagues reported that oral administration of NAC was capable of protecting the cochlea from loss of nonlinearity (the peculiar transductive mechanism of the inner ear that makes a wide dynamic range possible) from impulse noise (in this case, soldiers exposed to firearm discharge noise) (Lindblad et al. 2011). In contrast, Kramer et al. and Lin et al. have reported less successful attempts toward otoprotection from NAC administration in humans subject to noise (Kramer et al. 2006). Lin and colleagues also tested NAC as an otoprotectant after industrial noise exposure (Lin et al. 2011). This was a prospective, double-blind trial in male industrial workers exposed to 88–89 dBA noise with the intervention group receiving 1,200 mg a day of NAC for 2 weeks. This trial did not reveal any benefit from the use of the antioxidant. In both the Kramer and Lin studies, certain limitations could have disguised an effect. In the former, subjects were recruited who had been exposed to noise in a nightclub. Here, the consistency of exposure and wide variance in sensitivity of the subject could have been a confounder. It has been argued by Le Prell and her colleagues [who wrote an excellent review of the topic; (Le Prell et al. 2012)] that similar evaluations undertaken in a laboratory environment and with calibrated equipment might circumvent the methodological limitation and reveal an effect. In Lin’s report, Le Prell further argued that with a test–retest reliability of about 5 dB, an effect of attenuation in threshold shift (as reported by Lin et al.) of less than 3 dB would easily obscure the results. In addition, because these trials are relatively limited in scale, results can only be extrapolated with caution. Many commentators and investigators have expressed the need for larger human trials with potential otoprotectants like NAC (Scheck 2012). Several new studies have consolidated the reputation of NAC in protecting from NIHL. Dr. Kopke’s group in Oklahoma City has begun to report on an intriguing combination intervention where NAC is administered to noise-deafened animals along with a spin trap agent called HPN-07. Spin trap compounds were first noted to display synergism with NAC in attenuating NIHL by Choi et al. (2008). In the Kopke laboratory, 21 days of OBN (octave band noise) exposure was used to cause threshold shifts, and NAC was administered with the butylnitrone spin trap agent HPN-07. The report documented reduced ABR (auditory brainstem response) threshold shift in treated animals, lower DPOAE (distortion product otoacoustic emissions) shifts, and marked diminishment of hair cell loss in the basal cochlea (Lu et al. 2014).
d-methionine (a widely used free radical scavenger) has also often been used by investigators to assess its efficacy as an otoprotectant in NIHL. For example, Sampson and colleagues demonstrated that 400 mg/kg IP dosing of d-methionine was able to reverse noise-induced (4 kHz OBN; 100 dB SPL) oxidative stress in the mouse cochlea (measured as lipid peroxidase, SOD, and catalase activity) (Samson et al. 2008). Over many years, Campbell and colleagues have exhaustively detailed the protective role of d-methionine in the inner ear. In early work they reported that d-methionine attenuated damage to the stria vascularis in a cisplatin ototoxicity model (Campbell et al. 1999). In rats, her group demonstrated that this enzyme protected against cisplatin-induced ototoxicity, in part, by increasing the levels of antioxidant enzymes in the inner ear (Campbell et al. 2003). More recently, this laboratory has also shown that d-methionine can protect against noise-induced cochlear injury (Campbell et al. 2007) In chinchillas rendered permanently deaf with noise-induced hearing loss, NAC and d-methionine together showed effective protective synergy, while the two compounds acting alone did not (Clifford et al. 2011). More recently, dose-dependent protective effects of d-methionine have been reported in guinea pigs with NIHL (125–15 kHz, broad band white noise at 105 dB SPL) when the compound was administered at doses of 200, 400, and 600 mg/kg IP (Lo et al. 2013). At around the same time, another report from a laboratory that has generated a significant volume of d-methionine literature in NIHL described that when d-methionine was administered prior to noise exposure in chinchillas, hearing thresholds (measure by ABR recordings) were significantly improved (Claussen et al. 2013).
Other antioxidants have also been tested as potential otoprotectants in NIHL. These include vitamins A, C, and E, α-lipoic acid, and magnesium. Le Prell and colleagues published reports a few years ago showing that when more than one free radical scavenger was used in noise-induced hearing deficits in male guinea pigs, ABR thresholds were preserved. Due to variance, statistical power, and the designed mean intergroup difference the investigators had set for themselves, histological changes from noise trauma were less obvious between controls and treatment groups, and failed to reach statistical significance (Le Prell et al. 2007). Intriguingly, α-lipoic acid has also been shown to reduce carbon monoxide-induced potentiation of NIHL in rats (Pouyatos et al. 2008). Heinrich et al. also published data in a guinea pig NIHL model demonstrating that ascorbic acid treatment was able to reduce hearing thresholds after exposure to 90 dB SPL noise for 1 h. The authors also presented data on NO activity in the cochlea with ascorbic acid treatment. However, it was challenging to critique this report overall because the researchers failed to provide adequate detail of their NIHL paradigm (Heinrich et al. 2008). Le Prell has also compelled the notion of otoprotective dietary intervention on the principle of antioxidant interventions in NIHL. In their 2011 study, Le Prell et al. subject CBA/J mice to 8–16 kHz OBN noise for 2 h. Noise-naïve controls and noise-exposed animals were subject to ABR testing and micromorphometric evaluation of the cochlea with or without customized dietary manipulation (normal mouse chow supplemented with β-carotene, vitamin C, vitamin E, and magnesium). The study revealed a significant beneficial impact of the supplemented diet on ABR thresholds after noise exposure. While hair cell survival was not statistically improved, the number of type II fibrocytes in cochlear lateral wall was greater in animals fed the supplemented diet, and cell density of this type was found to be comparable to mice that had not been exposed to noise at all. There has been evidence from other studies that microstructural preservation of the type evident in these experiments correlates with ABR threshold preservation. Therefore, this finding has interesting implications (Mizutari et al. 2008; Le Prell et al. 2011). Other important work in subsequent years has also validated the role of α-lipoic acid as an antioxidant/otoprotectant in NIHL, either alone of as part of an enhanced diet in human studies. Prominent among these is Quaranta et al. who reported findings in normal human subjects who had been induced to have TTS after exposure to 90 dB of 3 kHz pure tone sounds for 10 min. Controls who received only the noise were compared with those who were subject to noise as well as preexposure oral treatment with α-lipoic acid (600 mg) and a third group who were given the same dose of the compound for 10 days prior to noise exposure. ABR thresholds of this third group (long-term preexposure prophylaxis) as well as the amplitude change of transient evoked otoacoustic emissions (TEOAEs) were lower in the long-term supplementation group compared to the other two groups (naïve controls and single-dose groups) (Quaranta et al. 2012). Additional signaling systems in the cochlea also mediate ROS-induced effects on cochlear physiology. For example, our work with the stilbenoid, resveratrol (a compound touted for its health benefits especially those from its presence in red wine), has shown that it attenuated the expression of cyclooxygenase-2 (COX-2) and concomitant ROS formation in the rat cochlea and that it reduces [it is more accurate] hearing threshold shift from acoustic trauma (Seidman et al. 2003, 2013). The basis of stable adhesion of leucocytes to the vascular endothelium is thought to be mediated at least in part by the expression of intercellular adhesion molecule-1 (ICAM-1), an inducible endothelial adhesive protein that serves as a counter-receptor for β2-integrins on leukocytes. Interaction of ICAM-1 with β2-integrins enables leukocytes to adhere firmly to the vascular endothelium and subsequently to migrate across the endothelial barrier. There is evidence that ICAM-1 expression, in general, can also promote ROS generation ubiquitously (Rahman and Fazal 2009). In view of this notion, our group also tested the possibility that immunochemical blockade of the ICAM-1 receptor might prove protective in NIHL via the deflation of ROS synthesis. We reported that intravenous administration of ICAM-1 antibody to Fischer rats successfully reduced the severity of hearing loss from broad band noise exposure (Seidman et al. 2009).
These are all compelling studies and have strongly advocated for expanding the evaluation of these promising antioxidants in human trials. Some limitations of animal studies apply in these examples and may be summarized as routes of administration that may not be possible in human and/or clinical settings and high experimental doses which pose challenges with respect to translation to the bedside. Finally, the bioavailability of antioxidants is varied and will need to be carefully determined in future studies.
4.6.1 GPx
In guinea pigs, the use of ebselen (which is a GPx mimic and inducer), when administered by gavage (mimicking the per oral route), has proven somewhat protective in TTS and PTS models of NIHL (Pourbakht and Yamasoba 2003; Lynch et al. 2004). Such studies are consistent with, and build on earlier evidence that GPx activity is notably decreased in NIHL and that engineered deletion of the GPx1 gene makes animals susceptible to NIHL (Ohlemiller et al. 1999, 2000). Specifically, Ebselen has been shown to diminish the severity of NIHL in rodent models with oral dosing (Pourbakht and Yamasoba 2003; Lynch et al. 2004). It has been postulated that because ebselen has been shown to block Cyt c release from the mitochondrion in cells that are stressed to the point of triggering the apoptotic cascade, the compound might exert beneficial effects on the cochlea via this mechanism as well (Boireau et al. 2000; Namura et al. 2001). Indeed, studies have compellingly demonstrated that ebselen can mitigate NIHL via its mimicry of GPx induction. Kil et al. found that GPx1 was a highly expressed isoform of GPx in the cochlea with concentrations in the rat organ of Corti, the spiral ganglion, the stria, and the spiral ligament. These investigators induced NIHL with 5 h of 113 dB noise (4–16 kHz) and administered ebselen at a dose of 4 mg/kg IP. The drug was able to reduce OHC loss secondary to noise exposure, minimize swelling of the stria, and boost GPx1 levels in the stria compared with controls (Kil et al. 2007). Yamasoba et al. have also published work that underscores ebselen’s protective role in NIHL. Their 2005 report looked at guinea pigs who had been exposed to 115 dB of OBN centered at 4 kHz. In these experiments, ebselen eliminated ABR threshold shifts and significantly tempered microanatomic changes from noise in the cochlea (swelling of afferent dendrites underneath IHCs (Yamasoba et al. 2005)). Recently, both phase I and II clinical trials with ebselen have completed and are being evaluated (Sound 2014; Lynch and Kil 2009).
4.6.2 Magnesium
Nearly 20 years ago, Attias et al. reported the finding that oral supplementation with magnesium (equivalent to 6.7 mM of Mg aspartate) was sufficient to afford partial protection from PTS 1 week after noise exposure (Attias et al. 1994). In contrast, a weak correlation was published between current serum levels of this mineral and the measured PTS in previously noise-exposed military combatants in a study by Walden and colleagues (Walden et al. 2000). This was interesting because on the one hand, magnesium supplementation appeared to offer protection in soldiers in terms of the severity of TTS. In a laboratory-based human subject study, no significant changes were noted in serum Mg levels between noise-exposed individuals and controls (Attias et al. 2004). Bearing in mind that Mg levels in the serum can be measured with difficulty and with less than desirable reliability, the relationship between magnesium supplementation and protection from NIHL, while provocative, needs to be investigated further. In fact there is now an extensive body of literature on the effects of Mg in the prevention of NIHL (for review, see Le Prell and Bao 2011). The mechanism of action of magnesium is also of interest as it is a potent glutamate antagonist and can mitigate glutamate excitotoxicity which may be implicated in NIHL (Mayer et al. 1984; Smith et al. 1989; Clerc et al. 2013).
4.6.3 N-Acetylcysteine
Continuing with the theme of military personnel who are exposed to noise, weapon training in the Marine Corps has been used as a test bed to determine the efficacy of NAC as an otoprotectant from noise. In 2003, a significantly sized trial by the US Department of the Navy enlisted 566 marine recruits who were undergoing weapon training with standard issue assault rifles. This was a randomized study with the treatment group receiving 900 mg of NAC with each meal through the length of the study. Possibly because all participants (experimental as well as control) were required to wear hearing protection, only about a third experienced measurable hearing loss. Of those who did, NAC was shown to have about a 25 % protective effect (modest by any standard) (Dolgin 2012). In contrast, under somewhat different circumstances, NAC was not able to exhibit protection in subjects who were exposed to loud sounds in a nightclub that had resulted in an average 14 dB TTS at 4 kHz (Kramer et al. 2005). In aggregate, the evidence does not clearly support NAC as a reliable protectant in NIHL.
4.6.4 Iron Chelators
A variety of iron chelating compounds can protect the cochlea by offering less iron to be available to react with H2O2, thereby limiting the Fenton reaction (iron-catalyzed hydrogen peroxide to yield hydroxyl radicals has been called Fenton’s reaction). In this class of compounds, deferoxamine (DFO) and dihydroxybenzoate (DHB) have both been used with some success in attenuating NIHL (Yamasoba et al. 1998). Iron-dependent ototoxic mechanisms in cisplatin-induced cochlear damage have also been investigated. When the iron chelator 2,2′-DPD (dipyridyl) was employed in guinea pig cochlear explants that had been exposed to cisplatin, significant rescue from cell death was observed (Rybak and Kelly 2003).
4.6.5 Trophic Factors
Brain-derived neurotrophic factor (BDNF) and glial cell-derived neurotrophic factor (GDNF) have also been used to rescue the cochlea from noise-induced injury (Shoji et al. 2000). The exact mechanism of these factors through which they purportedly provide protection, remains unclear. It is assumed, though not proven that they might have a role in upregulating antioxidant enzyme levels or alternatively in suppressing apoptotic triggers in the hair cells or spiral ganglion neurons (Jackson et al. 1990a, b; Perez-Polo et al. 1990; Pan and Perez-Polo 1993).
Overall, NIHL remains a significant public health problem. In some ways, an advancing technological society such as in the western hemisphere, the ubiquitous presence of environmental noise (e.g., industrial noise, and transportation noise) and occupational noise exposure (e.g., military, and civil aviation) challenges us to explore ways and means to ameliorate its health effects, including that on hearing. Much empirical work has identified the details of noise-induced hearing deficits. While many promising techniques have been discovered to protect the cochlea from the effects of noise, a single-agent protective regimen remains elusive. It is possible that multi-action modalities of treatment and prevention (such as synergistically acting drugs) will prove to be the mainstay of hearing protection from acoustic overstimulation. However, it is likely that significant advances will have to be made to achieve these goals.
4.7 The Use of Antioxidants in Aminoglycoside Toxicity
Rybak has described two strata of otoprotection in the context of aminoglycoside toxicity in the cochlea. These he has termed “upstream” (intervention that prevents the initial stages of lipid peroxidation at the hands of ROS) and “downstream” (interventions that can slow or reverse the process of cell death in the cochlea) (Rybak and Whitworth 2005). Between 1999 and 2000, investigators at a military medical university in China and the University of Michigan undertook a prospective, randomized, double-blind trial of aspirin supplementation in patients who were being treated with gentamicin for acute infections. This trial provided cogent evidence that an antioxidant can prove very useful in instances of aminoglycoside-induced ototoxicity. The trial showed that only 3 % of aspirin users suffered the consequence of hearing loss from gentamicin exposure. while hearing loss was noted in 13 % of those who were administered gentamicin with placebo (Sha et al. 2006; Chen et al. 2007).
4.8 Antioxidant Strategies in Aminoglycoside Ototoxicity: Upstream Protection
Several different drugs or compounds have been employed in attempts to block or mitigate early events in ROS-mediated aminoglycoside toxicity. These include vitamin E, d-methionine, α-lipoic acid, and ebselen (Takumida et al. 1999; Sha and Schacht 2000; Fetoni et al. 2003). Promising results were also recently reported for a combination of antioxidants including several vitamins and magnesium (Le Prell et al. 2014). In addition, iron chelators like deferoxamine have also been used with modest success (Dehne et al. 2002). More exotic ideas have also been tested, again with varying success, and these include modulating the role of NF-κB in the apoptotic cascade by using salicylate and 2,3-dihydroxybenzoate (Jiang et al. 2005), as well as an emerging report on nutrient supplementation in gentamicin ototoxicity (Le Prell et al. 2014). Polony and colleagues have found promising results with rasagiline (a selective monoamine oxidase type B inhibitor used in early Parkinson’s disease therapy) in aminoglycoside toxicity. The choice to test this drug was based on its known anti-apoptotic properties (by interfering with mitochondrial-dependent apoptotic signaling). In this paper a mouse model of kanamycin ototoxicity was used as a test bed, and the data demonstrated that at a dose of 3 mg/kg SC, rasagiline mitigated kanamycin-induced shifts in ABR thresholds in a dose-dependent manner. The investigators further explored potential mechanisms of this effect and found that the drug enhanced action potential-evoked dopamine release in the mouse cochlea (Polony et al. 2014). Chen et al. have also tested the mammalian peroxiredoxin, Prx3, as a mechanistic marker in a CBA/J mouse model of kanamycin toxicity. In their study, Prx3 was introduced into the cochlear explants via siRNA delivery with a hole drilled into the structure close to the round window via a microtube. The authors tested for the generation of free radicals in the hair cells in response to aminoglycoside exposure by immunofluorescent techniques. They then monitored changes in Prx3 expression in the hair cells in the context of gentamicin and kanamycin-induced hair cell loss. The data showed increased Prx3 expression in OHCs after about a week of kanamycin exposure. With loss of hair cells, there was a concomitant decrease in Prx3 levels as well. Further, these investigators used aminoglycoside-induced hair cell loss to test if Prx3 levels were correlated with antioxidant-mediated cell survival (using 2,3-dihydrobenzoate [DHB]). Interestingly, DHB treatment in kanamycin-induced cochlear injury also preserved the level of expression of Prx3 (Chen et al. 2013). Coffin et al. have also recently demonstrated that cell death pathways in hair cells in ototoxicity from aminoglycosides are more specific than previously assumed. For example, in Coffin’s zebra fish lateral line model of ototoxicity, neomycin, gentamicin, and cisplatin induced or triggered a distinct subset of the programmed cell death machinery. This was evident from the differing response this model exhibited when exposed to specific protective interventions. A proteasome inhibitor protected the hair cells from either aminoglycoside or cisplatin toxicity. d-Methionine, on the other hand, protected from gentamicin and cisplatin effects but not from neomycin toxicity (Sha and Schacht 2000; Campbell et al. 2007; Coffin et al. 2013).
Neurotrophic growth factors have been tried, and these have displayed their ability to bolster antioxidant enzyme activity, reduce the formation of NO [thereby attenuating the involvement of RNS], and increase the anti-apoptotic Bcl-2 proteins in the noise-exposed cochlea (Rybak and Whitworth 2005). Glucocorticoids like dexamethasone have been used to protect isolated OHCs from aminoglycoside toxicity; however, the exact mechanism is unclear. Again, it is presumed that steroids function to inhibit NO and diminish ROS synthesis (Rybak and Whitworth 2005). In addition, certain experimental compounds designed to augment endogenous antioxidant enzymes have seen recent favor, and they include the SOD mimetic M40403 which has exhibited protection of organotypic OHC culture exposed to gentamicin (Nicotera et al. 2004). Interestingly, in a guinea pig model of aminoglycoside toxicity, adenoviral vectoring of SOD1, SOD2, and catalase was partially able to protect the inner ear from toxicity of aminoglycosides (Kawamoto et al. 2004).
Non-pharmaceutic modalities have also been employed in aminoglycoside ototoxicity research. These include “cell toughening” (preexposing the cochlea to low levels of toxic stress to eventually tolerate higher levels of the drugs) has been shown to upregulate the level of antioxidant enzymes in the cochlea and specifically in the guinea pig; the use of amikacin in this manner has been successful (Jacono et al. 1998; Ding et al. 2003). While loop diuretics are known to display synergism with aminoglycosides with regard to ototoxicity, delayed administration of ethacrynic acid has been shown to reduce the level of aminoglycosides in the perilymph with concomitant reduction in cochlear damage (Ding et al. 2003).
4.8.1 Antioxidant Strategies in Aminoglycoside Ototoxicity: Downstream Protection
With the emerging possibility that apoptosis may not be irreversible once initiated, investigators have tested methods to block early apoptosis promoters such as GTPases, Rho, and Rac. One such candidate is toxin B of Clostridium difficile (C. difficile) which has been reported to provide dose-dependent protection from aminoglycosides (Bodmer et al. 2002). Further, the inhibition of the JNK signaling cascade has also been shown to attenuate aminoglycoside effects in the cochlea (Abi-Hachem et al. 2010). Another similar compound, D-JNK-1, has shown positive results in neomycin toxicity (Wang et al. 2003b; Minami et al. 2004).
Further downstream, blockade of the release of Cyt c from the mitochondrion with the tetracycline antibiotic minocycline has also reportedly yielded positive data in vitro (Corbacella et al. 2004). It has also been shown that caspase inhibitors combined with a p38 MAPK inhibitor afford synergistic protection in models of gentamicin toxicity (Wei et al. 2005).
In summary, while aminoglycoside antimicrobials are very useful as therapeutic agents against serious gram-negative infections in clinical practice, their potential for cochlear injury and their ability to sensitize the inner ear to noise-induced damage make them agents that must be used with great care. About a third of subjects who receive aminoglycosides will experience adverse effects and their slow clearance from cochlear fluids compounds their toxic profile (Campo et al. 2013). These issues are examined and discussed in much greater detail in Chap. 12 of this volume.
4.9 Antioxidant Strategies in Cisplatin Ototoxicity
Cisplatin’s clinical usefulness is seriously compromised by its associated nephrotoxicity, neurotoxicity, and ototoxicity. Over the previous few decades, more and more evidence has emerged that cisplatin ototoxicity is mediated via the overproduction of or underprotection from ROS (Clerici et al. 1996). In animal models cisplatin administration has been proven to deplete GPx, SOD, catalase, and glutathione reductase (Rybak et al. 2000). The isoform of NADPH oxidase (a membrane bound complex and important source of superoxide), NOX3, is preferentially expressed in the inner ear, and in this location it is also an important source of ROS synthesis. Studies in non-cochlear tissue have implicated NOX3 as being responsible for superoxide production in response to cisplatin (in HEK293 cells) (Banfi et al. 2004). This implies the possibility that the enzyme could be tightly targeted to protect from cisplatin toxicity (Rybak and Whitworth 2005). It is not inconceivable that specific inhibition of Nox3 might achieve protection in the cochlea, and in other contexts, several such compounds are being investigated (Schramm et al. 2012). Antioxidant strategies in the case of cisplatin, like aminoglycosides described above, can also be viewed in upstream and downstream contexts.
With cisplatin toxicity, pharmacologic compounds containing thiol groups have been widely tested based on the logic that thiols are potent reducing agents to countermand the impact of oxidative stress. Thus, sodium thiosulfate, d– and l-methionine, diethyldithiocarbamate, methylthiobenzoic acid, lipoic acid, NAC, tiopronin, glutathione ester, and amifostine have reduced cisplatin ototoxicity (Rybak and Whitworth 2005). Sodium thiosulfate perfusion into the guinea pig cochlea, for example, was shown by Wang and colleagues to protect from cisplatin-induced damage (Wang et al. 2003a). When rats were pretreated with d-methionine and then exposed to cisplatin, auditory thresholds and hair cell populations were preserved and antioxidant enzyme depletion was minimized (Campbell et al. 2003). In hamsters, similar methods with amifostine also proved effective (Church et al. 2004).
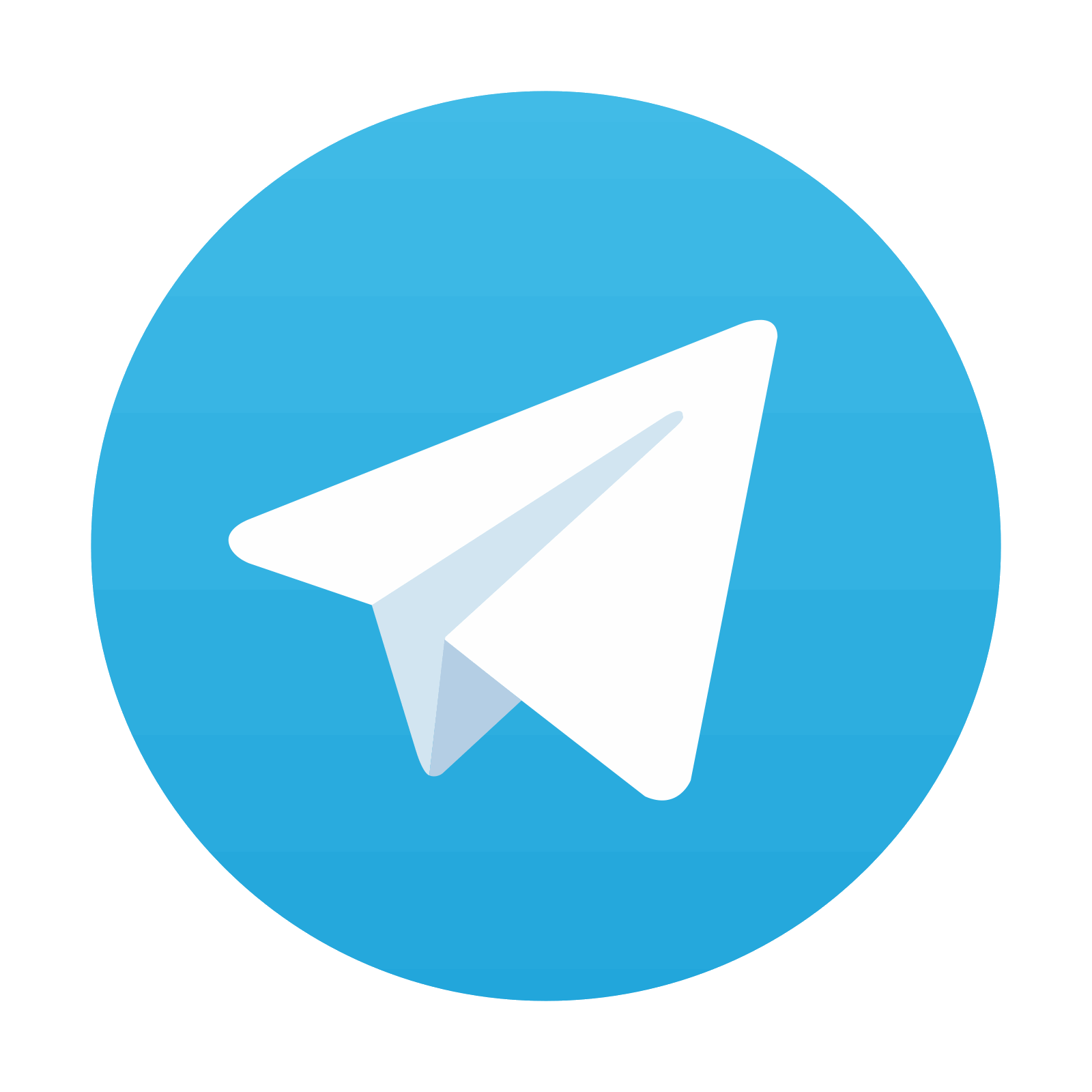
Stay updated, free articles. Join our Telegram channel
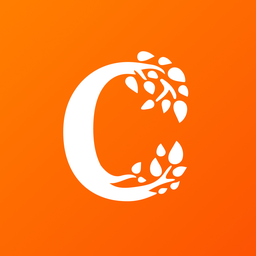
Full access? Get Clinical Tree
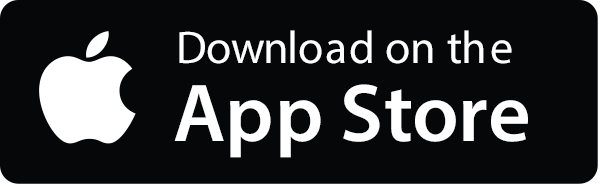
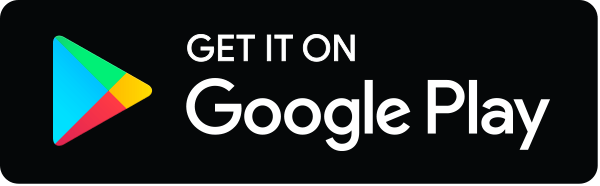