INTRODUCTION
Drugs have been used for the treatment of infectious diseases since the 17th century (eg, quinine for malaria, emetine for amebiasis); however, chemotherapy as a science began in the first decade of the 20th century with understanding of the principles of selective toxicity, the specific chemical relationships between microbial pathogens and drugs, the development of drug resistance, and the role of combined therapy. Experiments led to the arsphenamines for syphilis, the first planned chemotherapeutic regimen.
The current era of antimicrobial chemotherapy began in 1935 with the discovery of the sulfonamides. In 1940, it was demonstrated that penicillin, discovered in 1929, could be an effective therapeutic substance. During the next 25 years, research on chemotherapeutic agents centered largely on substances of microbial origin called antibiotics. The isolation, concentration, purification, and mass production of penicillin were followed by the development of streptomycin, tetracyclines, chloramphenicol, and many other agents. These substances were originally isolated from filtrates of media in which their respective molds had grown. Synthetic modification of previously described drugs has been prominent in the development of new antimicrobial agents.
Antimicrobial agents commonly used in the treatment of patients with bacterial infections are presented in this chapter. The chemotherapy of viruses, fungi, and parasites is discussed in Chapters 30, 45, and 46, respectively. Additional comments on antimicrobial susceptibility testing for bacteria are found in Chapter 47.
MECHANISMS OF ACTION OF ANTIMICROBIAL DRUGS
Antimicrobial drugs act in one of several ways: by selective toxicity, by inhibition of cell membrane synthesis and function, by inhibition of protein synthesis, or by inhibition of nucleic acid synthesis.
An ideal antimicrobial agent exhibits selective toxicity, which means that the drug is harmful to a pathogen without being harmful to the host. Often, selective toxicity is relative rather than absolute; this implies that a drug in a concentration tolerated by the host may damage an infecting microorganism.
Selective toxicity may be a function of a specific receptor required for drug attachment, or it may depend on the inhibition of biochemical events essential to the pathogen but not to the host. The mechanisms of action of antimicrobial drugs can be discussed under four headings:
Inhibition of cell wall synthesis
Inhibition of cell membrane function
Inhibition of protein synthesis (ie, inhibition of translation and transcription of genetic material)
Inhibition of nucleic acid synthesis
Bacteria have a rigid outer layer, the cell wall. The cell wall maintains the shape and size of the microorganism, which has a high internal osmotic pressure. Injury to the cell wall (eg, by lysozyme) or inhibition of its formation may lead to lysis of the cell. In a hypertonic environment (eg, 20% sucrose), damaged cell wall formation leads to formation of spherical bacterial “protoplasts” from gram-positive organisms or “spheroplasts” from gram-negative organisms; these forms are limited by the fragile cytoplasmic membrane. If such protoplasts or spheroplasts are placed in an environment of ordinary tonicity, they take up fluid rapidly, swell, and may explode. Specimens from patients being treated with cell wall–active antibiotics often show swollen or misshapen bacteria.
The cell wall contains a chemically distinct complex polymer “mucopeptide” (“peptidoglycan”) consisting of polysaccharides and a highly cross-linked polypeptide. The polysaccharides regularly contain the amino sugars N-acetylglucosamine and acetylmuramic acid. The latter is found only in bacteria. To the amino sugars are attached short peptide chains. The final rigidity of the cell wall is imparted by cross-linking of the peptide chains (eg, through pentaglycine bonds) as a result of transpeptidation reactions carried out by several enzymes. The peptidoglycan layer is much thicker in the cell wall of gram-positive than of gram-negative bacteria.
All β-lactam drugs are selective inhibitors of bacterial cell wall synthesis and therefore active against growing bacteria. This inhibition is only one of several different activities of these drugs, but it is the best understood. The initial step in drug action consists of binding of the drug to cell receptors (penicillin-binding proteins [PBPs]). There are at least six different PBPs (molecular weight [MW], 40–120 kilodaltons [kD]), some of which are transpeptidation enzymes. Different receptors have different affinities for a drug, and each may mediate a different effect. For example, attachment of penicillin to one PBP may result chiefly in abnormal elongation of the cell, but attachment to another PBP may lead to a defect in the periphery of the cell wall with resulting cell lysis. PBPs are under chromosomal control, and mutations may alter their number or their affinity for β-lactam drugs.
After a β-lactam drug has attached to one or more receptors, the transpeptidation reaction is inhibited, and peptidoglycan synthesis is blocked. The next step probably involves removal or inactivation of an inhibitor of autolytic enzymes in the cell wall. This activates the lytic enzyme and results in lysis if the environment is isotonic. In a markedly hypertonic environment, the microbes change to protoplasts or spheroplasts, covered only by the fragile cell membrane. In such cells, synthesis of proteins and nucleic acids may continue for some time.
The inhibition of the transpeptidation enzymes by penicillins and cephalosporins may be attributable to a structural similarity of these drugs to acyl-d-alanyl-d-alanine. The transpeptidation reaction involves loss of a d-alanine from the pentapeptide.
The remarkable lack of toxicity of β-lactam drugs to mammalian cells must be attributed to the absence in animal cells of a bacterial type cell wall with its peptidoglycan. The difference in susceptibility of gram-positive and gram-negative bacteria to various penicillins or cephalosporins probably depends on structural differences in their cell walls (eg, amount of peptidoglycan, presence of receptors and lipids, nature of cross-linking, activity of autolytic enzymes) that determine penetration, binding, and activity of the drugs.
Resistance to penicillins may be determined by the organism’s production of penicillin-destroying enzymes (β-lactamases). The β-lactamases open the β-lactam ring of penicillins and cephalosporins and abolish their antimicrobial activity. β-Lactamases have been described for many species of gram-positive and gram-negative bacteria. Some β-lactamases are plasmid mediated (eg, penicillinase of Staphylococcus aureus), and others are chromosomally mediated (eg, many species of gram-negative bacteria). All of the plasmid-mediated β-lactamases are produced constitutively and have a high propensity to move from one species of bacteria to another (eg, β-lactamase–producing Neisseria gonorrhoeae, Haemophilus influenzae, and enterococci). Chromosomally mediated β-lactamases may be constitutively produced (eg, Bacteroides, Acinetobacter species), or they may be inducible (eg, Enterobacter, Citrobacter, Pseudomonas species).
There is one group of β-lactamases that is occasionally found in certain species of gram-negative bacilli, usually Klebsiella pneumoniae and Escherichia coli. These enzymes are termed extended-spectrum β-lactamases (ESBLs) because they confer upon the bacteria the additional ability to hydrolyze the β-lactam rings of cefotaxime, ceftazidime, or aztreonam.
The classification of β-lactamases is complex, based on the genetics, biochemical properties, and substrate affinity for a β-lactamase inhibitor (clavulanic acid) (Table 28-1 has the two major classification systems). Clavulanic acid, sulbactam, and tazobactam are β-lactamase inhibitors that have a high affinity for and irreversibly bind some β-lactamases (eg, penicillinase of S aureus) but are not hydrolyzed by the β-lactamase. These inhibitors protect simultaneously present hydrolyzable penicillins (eg, ampicillin, amoxicillin, and ticarcillin) from destruction. Certain penicillins (eg, cloxacillin) also have a high affinity for β-lactamases.
Bush-Jacoby-Medeiros System Group | Enzyme Type | Inhibition by Clavulanate | Ambler System | Main Attributes |
---|---|---|---|---|
1 | Cephalosporinase | No | C | Chromosomal; AMP-C type enzymes; resistant to all β-lactams except carbapenems |
2a | Penicillinase | Yes | A (serine) | Staphylococcal penicillinases; Bacillus cereus |
2b | Broad-spectrum | Yes | A | TEM-1, TEM-2, SHV-1 |
2be | Extended-spectrum | Yes | A (serine) | TEM- and SHV variants; CTX-M-derived; GES-1, 2; VEB-1, 2 |
2br | Inhibitor resistant | Diminished | A | Inhibitor resistant TEM-30 |
2c | Carbenicillinase | Yes | A | Carbenicillin hydrolyzing |
2d | Cloxacillinase | Yes | D* or A | Oxacillin hydrolyzing (OXA) |
2e | Cephalosporinase | Yes | A | Cephalosporinases |
2f | Carbapenemase | Yes | A | Carbapenemases inhibited by clavulanate (eg, IMI, KPC, SME-1) |
3 | Metalloenzymes | No | B (Zn2+) | Zinc-dependent carbapenemases (eg, IMP, VIM, NDM-1, GIM, SPM, SIM) |
4 | Penicillinase | No | Not classified | Misc enzymes |
Shortly after their first description almost 3 decades ago, the most common ESBLs were of the class A TEM and SHV plasmid-mediated types (see Table 28-1). Currently throughout much of the world, the CTX-M enzymes have become more prevalent. These enzymes are more active against cefotaxime and ceftriaxone than ceftazidime and seem to be inhibited more readily by tazobactam than the other β-lactamase inhibitors. Of most concern is the emergence of K pneumoniae carbapenemases (KPC), which are ESBL-type enzymes that confer resistance to third- and fourth-generation cephalosporins and carbapenems. This resistance mechanism is plasmid mediated and has spread nosocomially among many hospitals throughout the United States and other countries.
Although they were discovered in the mid-1960s, global spread of genes encoding metallo-β-lactamases has facilitated spread of these broad-range, inhibitor-resistant enzymes among many gram-negative pathogens. This has ushered in an era of widespread dissemination of carbapenem-resistant Enterobacteriaceae possessing the VIM-type (Verona integron-encoded metallo-β-lactamase) and NDM-type (New Delhi metallo-β-lactamase) of these enzymes. VIM-type enzymes first appeared in Pseudomonas aeruginosa and Acinetobacter baumannii, but within the past decade, they have spread to Enterobacteriaceae. There are more than 20 types, and they are most prevalent in Europe, the Middle East, and Asia. NDM-1 is relatively new, having been described first in a K pneumoniae strain in Sweden from a patient who had traveled to India. In addition to spread to other Enterobacteriaceae, reports of NDM-1 producing A baumannii have also appeared. Because these organisms often contain genes that encode resistance to other classes of antimicrobials, such as fluoroquinolones and aminoglycosides, options for treatment are very limited to agents such colistin. Therefore, such patients are often placed on maximum infection control precautions to prevent spread to other patients within hospital environments.
There are two other types of resistance mechanisms. One is caused by the absence of some PBPs and occurs as a result of chromosomal mutation; the other results from failure of the β-lactam drug to activate the autolytic enzymes in the cell wall. As a result, the organism is inhibited but not killed. Such tolerance has been observed especially with staphylococci and certain streptococci.
Examples of agents acting by inhibition of cell wall synthesis are penicillins, the cephalosporins, vancomycin and other glycopeptide analogues such as teicoplanin and the newer lipoglycopeptides, and cycloserine. Several other drugs, including bacitracin, teicoplanin, vancomycin, ristocetin, and novobiocin, inhibit early steps in the biosynthesis of the peptidoglycan. Because the early stages of synthesis take place inside the cytoplasmic membrane, these drugs must penetrate the membrane to be effective.
The cytoplasms of all living cells are bounded by the cytoplasmic membrane, which serves as a selective permeability barrier and carries out active transport functions and thus controls the internal composition of the cell. If the functional integrity of the cytoplasmic membrane is disrupted, macromolecules and ions escape from the cell, and cell damage or death ensues. The cytoplasmic membrane of bacteria and fungi has a structure different from that of animal cells and can be more readily disrupted by certain agents. Consequently, selective chemotherapy is possible.
Detergents, which contain lipophilic and hydrophilic groups, disrupt cytoplasmic membranes and kill the cell (see Chapter 4). One class of antibiotics, the polymyxins, consists of detergent-like cyclic peptides that selectively damage membranes containing phosphatidylethanolamine, a major component of bacterial membranes. A number of antibiotics specifically interfere with biosynthetic functions of the cytoplasmic membranes (eg, nalidixic acid and novobiocin inhibit DNA synthesis, and novobiocin also inhibits teichoic acid synthesis).
A third class of membrane-active agents is the ionophores, compounds that permit rapid diffusion of specific cations through the membrane. Valinomycin, for example, specifically mediates the passage of potassium ions. Some ionophores act by forming hydrophilic pores in the membrane; others act as lipid-soluble ion carriers that behave as though they shuttle back and forth within the membrane. Ionophores can kill cells by discharging the membrane potential, which is essential for oxidative phosphorylation, as well as for other membrane-mediated processes; they are not selective for bacteria but act on the membranes of all cells.
Daptomycin is a cyclic 13-member lipopeptide antibiotic that is rapidly bactericidal by binding to the cell membrane in a calcium ion–dependent manner, causing depolarization of bacterial membrane potential. This leads to intracellular potassium release that causes cell death. Currently, this agent is approved for use in the treatment of S aureus bloodstream infections and skin and soft tissue infections caused by gram-positive bacteria, particularly organisms that are highly resistant to β-lactam agents and vancomycin.
Telavancin, one of the newer lipoglycopeptides, is also capable of depolarizing the cell membranes of staphylococci by a poorly understood mechanism.
Other examples of agents acting by inhibition of cell membrane function are amphotericin B, colistin, and the imidazoles and triazoles.
It is established that macrolides, lincosamides, tetracyclines, glycylcyclines, aminoglycosides, and chloramphenicol can inhibit protein synthesis in bacteria. The precise mechanisms of action differ among these classes of drugs.
Whereas bacteria have 70S ribosomes, mammalian cells have 80S ribosomes. The subunits of each type of ribosome, their chemical composition, and their functional specificities are sufficiently different to explain why antimicrobial drugs can inhibit protein synthesis in bacterial ribosomes without having a major effect on mammalian ribosomes.
In normal microbial protein synthesis, the mRNA message is simultaneously “read” by several ribosomes that are strung out along the mRNA strand. These are called polysomes.
The mode of action of streptomycin has been studied far more intensively than that of other aminoglycosides, but all act similarly. The first step is the attachment of the aminoglycoside to a specific receptor protein (P 12 in the case of streptomycin) on the 30S subunit of the microbial ribosome. Second, the aminoglycoside blocks the normal activity of the “initiation complex” of peptide formation (mRNA + formyl methionine + tRNA). Third, the mRNA message is misread on the “recognition region” of the ribosome; consequently, the wrong amino acid is inserted into the peptide, resulting in a nonfunctional protein. Fourth, aminoglycoside attachment results in the breakup of polysomes and their separation into monosomes incapable of protein synthesis. These activities occur more or less simultaneously, and the overall effect is usually an irreversible event—killing of the bacterium.
Chromosomal resistance of microbes to aminoglycosides principally depends on the lack of a specific protein receptor (modification of the target site caused by mutations) on the 30S subunit of the ribosome. Plasmid-dependent resistance to aminoglycosides depends on the production by the microorganism of adenylating, phosphorylating, or acetylating enzymes that destroy the drugs (most common mechanism). A third type of resistance consists of a “permeability defect,” an outer membrane change that reduces active transport of the aminoglycoside into the cell so the drug cannot reach the ribosome. Often this is plasmid mediated.
These drugs (erythromycins, azithromycin, clarithromycin, and roxithromycin and the ketolide telithromycin) bind to the 50S subunit of the ribosome, and the binding site is domain V of the 23S rRNA. They may interfere with formation of initiation complexes for peptide chain synthesis or may interfere with aminoacyl translocation reactions. Some macrolide-resistant bacteria lack the proper receptor on the ribosome (through methylation of the 23S rRNA target site). The erm (erythromycin ribosome methylation) genes that encode this mechanism may be under plasmid or chromosomal control. They may be expressed constitutively or may be induced by subinhibitory concentrations of macrolides. Other less common mechanisms of resistance include production of inactivating enzymes or mef– and msr-encoded efflux. Efflux-mediated resistance does not affect susceptibility to ketolides.
Clindamycin and lincomycin bind to the 50S subunit of the microbial ribosome and resemble macrolides in binding site, antibacterial activity, and mode of action. Chromosomal mutants are resistant because they lack the proper binding site on the 50S subunit.
Tetracyclines bind reversibly to the 30S subunit of microbial ribosomes. They inhibit protein synthesis by blocking the attachment of charged aminoacyl-tRNA. Thus, they prevent introduction of new amino acids to the nascent peptide chain. The action is usually inhibitory and reversible upon withdrawal of the drug. Resistance to tetracyclines occurs by multiple mechanisms—efflux, ribosomal protection proteins, and chemical modification, among others.
The first two are the most important and occur as follows: Efflux pumps, located in the bacterial cell cytoplasmic membrane, are responsible for pumping the drug out of the cell. Tet gene products are responsible for protecting the ribosome, likely through mechanisms that induce conformational changes. These conformational changes either prevent binding of the tetracyclines or cause their dissociation from the ribosome. This is often plasmid controlled. Mammalian cells do not actively concentrate tetracyclines.
The glycylcyclines are synthetic analogues of the tetracyclines. The agent that is available for use in the United States and Europe is tigecycline, a derivative of minocycline. The glycylcyclines inhibit protein synthesis in a manner similar to the tetracyclines but they demonstrate more avid binding to the ribosome. Tigecycline is active against a broad range of gram-positive and gram-negative bacteria, including strains resistant to the typical tetracyclines. This drug has approved indications for treatment of skin and skin structure infections, in intra-abdominal infections and for the treatment of community-acquired pneumonia, particularly those caused by bacterial pathogens resistant to a variety of other antimicrobial agents. In addition, use of this drug for treatment of multidrug-resistant health care–associated infections (except P aeruginosa) has substantially increased. In 2013, the FDA issued a warning based upon an analysis of 13 clinical trials that demonstrated an increased risk of death with tigecycline (see ). It is suggested that this drug be reserved for situations where other agents are not available or cannot be used because of resistance.
Chloramphenicol binds to the 50S subunit of the 70S bacterial ribosome. It interferes with the binding of new amino acids to the nascent peptide chain, largely because chloramphenicol inhibits peptidyl transferase. Chloramphenicol is mainly bacteriostatic, and growth of microorganisms resumes when the drug is withdrawn. Microorganisms resistant to chloramphenicol usually produce the chloramphenicol acetyltransferases, which destroys drug activity. The production of this enzyme is usually under the control of plasmid-mediated resistance genes called cat genes. Other mechanisms of resistance include efflux pumps and decreased membrane permeability.
Quinupristin–dalfopristin is a combination of two pristinamycin derivatives. These two agents act synergistically to achieve bactericidal activity against gram-positive bacteria not seen with either agent alone. The mechanism of action appears to be irreversible binding to different sites on the 50S subunits of the 70S bacterial ribosomes. Resistance may occur from conformational changes in the target, efflux, and enzymatic inactivation.
The oxazolidinones possess a unique mechanism of inhibition of protein synthesis primarily in gram-positive bacteria. These compounds interfere with translation by inhibiting the formation of N-formylmethionyl-tRNA, the initiation complex at the 23S ribosome. Linezolid was the first agent to become commercially available and it has seen widespread usage in the treatment of a variety of serious gram-positive infections including those caused by vancomycin resistant enterococci and even mycobacterial infections.
Examples of drugs acting by inhibition of nucleic acid synthesis are the quinolones, pyrimethamine, rifampin, sulfonamides, trimethoprim, and trimetrexate. Rifampin inhibits bacterial growth by binding strongly to the DNA-dependent RNA polymerase of bacteria. Thus, it inhibits bacterial RNA synthesis. Rifampin resistance results from a change in RNA polymerase because of a chromosomal mutation that occurs with high frequency. The mechanism of rifampin action on viruses is different. It blocks a late stage in the assembly of poxviruses.
All quinolones and fluoroquinolones inhibit microbial DNA synthesis by blocking DNA gyrases, topoisomerase enzymes that play key roles in DNA replication and repair.
For many microorganisms, p-aminobenzoic acid (PABA) is an essential metabolite. The specific mode of action of PABA involves an adenosine triphosphate (ATP)–dependent condensation of a pteridine with PABA to yield dihydropteroic acid, which is subsequently converted to folic acid. PABA is involved in the synthesis of folic acid, an important precursor to the synthesis of nucleic acids. Sulfonamides are structural analogs of PABA and inhibit dihydropteroate synthetase.
Sulfonamides can enter into the reaction in place of PABA and compete for the active center of the enzyme. As a result, nonfunctional analogs of folic acid are formed, preventing further growth of the bacterial cell. The inhibiting action of sulfonamides on bacterial growth can be counteracted by an excess of PABA in the environment (competitive inhibition). Animal cells cannot synthesize folic acid and must depend on exogenous sources. Some bacteria, similar to animal cells, are not inhibited by sulfonamides. Many other bacteria, however, synthesize folic acid, as mentioned earlier, and consequently are susceptible to action by sulfonamides.
Trimethoprim (3,4,5-trimethoxybenzylpyrimidine) inhibits dihydrofolic acid reductase 50,000 times more efficiently in bacteria than in mammalian cells. This enzyme reduces dihydrofolic to tetrahydrofolic acid, a stage in the sequence leading to the synthesis of purines and ultimately of DNA. Sulfonamides and trimethoprim each can be used alone to inhibit bacterial growth. If used together, they produce sequential blocking, resulting in a marked enhancement (synergism) of activity. Such mixtures of sulfonamide (five parts) plus trimethoprim (one part) have been used in the treatment of pneumocystis pneumonia, malaria, shigella enteritis, systemic salmonella infections, urinary tract infections, and many others.
Pyrimethamine also inhibits dihydrofolate reductase, but it is more active against the enzyme in mammalian cells and therefore is more toxic than trimethoprim. Pyrimethamine plus sulfonamide or clindamycin is the current treatment of choice in toxoplasmosis and some other protozoal infections.
RESISTANCE TO ANTIMICROBIAL DRUGS
There are many different mechanisms by which microorganisms might exhibit resistance to drugs.
Microorganisms produce enzymes that destroy the active drug. Examples: Staphylococci resistant to penicillin G produce a β-lactamase that destroys the drug. Other β-lactamases are produced by gram-negative rods (see Table 28-1). Gram-negative bacteria resistant to aminoglycosides (by virtue of a plasmid) produce adenylating, phosphorylating, or acetylating enzymes that destroy the drug.
Microorganisms change their permeability to the drug. Examples: Tetracyclines accumulate in susceptible bacteria but not in resistant bacteria. Resistance to polymyxins is also associated with a change in permeability to the drugs. Streptococci have a natural permeability barrier to aminoglycosides. This can be partly overcome by the simultaneous presence of a cell wall–active drug such as a penicillin. Resistance to amikacin and to some other aminoglycosides may depend on a lack of permeability to the drugs caused by an outer membrane change that impairs active transport into the cell.
Microorganisms develop an altered structural target for the drug (see also 5). Examples: Erythromycin-resistant organisms have an altered receptor on the 50S subunit of the ribosome, resulting from methylation of a 23S ribosomal RNA. Resistance to some penicillins and cephalosporins may be a function of the loss or alteration of PBPs. Penicillin resistance in Streptococcus pneumoniae and enterococci is attributable to altered PBPs.
Microorganisms develop an altered metabolic pathway that bypasses the reaction inhibited by the drug. Example: Some sulfonamide-resistant bacteria do not require extracellular PABA but, similar to mammalian cells, can use preformed folic acid.
Microorganisms develop an altered enzyme that can still perform its metabolic function but is much less affected by the drug. Example: In trimethoprim-resistant bacteria, the dihydrofolic acid reductase is inhibited far less efficiently than in trimethoprim-susceptible bacteria.
Microorganisms can develop efflux pumps that transport the antibiotics out of the cell. Many gram positive and especially gram-negative organisms have developed this mechanism for tetracyclines (common), macrolides, fluoroquinolones, and even β-lactam agents.
Active replication of bacteria is required for most antibacterial drug actions. Consequently, microorganisms that are metabolically inactive (nonmultiplying) may be phenotypically resistant to drugs. However, their offspring are fully susceptible. Example: Mycobacteria often survive in tissues for many years after infection yet are restrained by the host’s defenses and do not multiply. Such “persisting” organisms are resistant to treatment and cannot be eradicated by drugs. Yet if they start to multiply (eg, after suppression of cellular immunity in the patient), they are fully susceptible to the same drugs.
Microorganisms may lose the specific target structure for a drug for several generations and thus be resistant. Example: Penicillin-susceptible organisms may change to cell wall–deficient L forms during penicillin administration. Lacking cell walls, they are resistant to cell wall–inhibitor drugs (penicillins, cephalosporins) and may remain so for several generations. When these organisms revert to their bacterial parent forms by resuming cell wall production, they are again susceptible to penicillin.
Microorganisms may infect the host at sites where antimicrobials are excluded or are not active. Examples: Aminoglycosides such as gentamicin are not effective in treating Salmonella enteric fevers because the salmonellae are intracellular and the aminoglycosides do not enter the cells. Similarly, only drugs that enter cells are effective in treating Legionnaires’ disease because of the intracellular location of Legionella pneumophila.
Most drug-resistant microbes emerge as a result of genetic change and subsequent selection processes by antimicrobial drugs.
This develops as a result of spontaneous mutation in a locus that controls susceptibility to a given antimicrobial drug. The presence of the antimicrobial drug serves as a selecting mechanism to suppress susceptible organisms and favor the growth of drug-resistant mutants. Spontaneous mutation occurs with a frequency of 10−12–10−7 and thus is an infrequent cause of the emergence of clinical drug resistance in a given patient. However, chromosomal mutants resistant to rifampin occur with high frequency (~10−7–105). Consequently, treatment of bacterial infections with rifampin as the sole drug often fails. Chromosomal mutants are most commonly resistant by virtue of a change in a structural receptor for a drug. Thus, the P 12 protein on the 30S subunit of the bacterial ribosome serves as a receptor for streptomycin attachment. Mutation in the gene controlling that structural protein results in streptomycin resistance. Mutation can also result in the loss of PBPs, making such mutants resistant to β-lactam drugs.
Bacteria often contain extrachromosomal genetic elements called plasmids. Their features are described in Chapter 7.
Some plasmids carry genes for resistance to one—and often several—antimicrobial drugs. Plasmid genes for antimicrobial resistance often control the formation of enzymes capable of destroying the antimicrobial drugs. Thus, plasmids determine resistance to penicillins and cephalosporins by carrying genes for the formation of β-lactamases. Plasmids code for enzymes that acetylate, adenylate, or phosphorylate various aminoglycosides; for enzymes that determine the active transport of tetracyclines across the cell membrane; and for others.
Genetic material and plasmids can be transferred by transduction, transformation, and conjugation. These processes are discussed in Chapter 7.
Microorganisms resistant to a certain drug may also be resistant to other drugs that share a mechanism of action. Such relationships exist mainly between agents that are closely related chemically (eg, different aminoglycosides) or that have a similar mode of binding or action (eg, macrolides and lincosamides). In certain classes of drugs, the active nucleus of the chemical is so similar among many congeners (eg, tetracyclines) that extensive cross-resistance is to be expected.
Emergence of drug resistance in infections may be minimized in the following ways: (1) by maintaining sufficiently high levels of the drug in the tissues to inhibit both the original population and first-step mutants; (2) by simultaneously administering two drugs that do not give cross-resistance, each of which delays the emergence of mutants resistant to the other drug (eg, rifampin and isoniazid [INH] in the treatment of tuberculosis); and (3) by avoiding exposure of microorganisms to a particularly valuable drug by limiting its use, especially in hospitals.
A few examples will illustrate the impact of the emergence of drug-resistant organisms and their selection by the widespread use of antimicrobial drugs.
When sulfonamides were first used in the late 1930s for the treatment of gonorrhea, virtually all isolates of gonococci were susceptible, and most infections were cured. A few years later, most strains had become resistant to sulfonamides, and gonorrhea was rarely curable by these drugs. Most gonococci were still highly susceptible to penicillin. Over the next decades, there was a gradual increase in resistance to penicillin, but large doses of that drug were still curative. In the 1970s, β-lactamase–producing gonococci appeared, first in the Philippines and in West Africa, and then spread to form endemic foci worldwide. Such infections could not be treated effectively by penicillin but were treated with spectinomycin. Resistance to spectinomycin has appeared. Third-generation cephalosporins or quinolones were recommended to treat gonorrhea. However, the emergence of quinolone resistance in some geographic locations has subsequently limited their use, and they are no longer recommended as first-line treatment. Of more concern are the recent observations regarding treatment failures with oral third-generation cephalosporins due to rising MICs among N gonorrhoeae. Parenteral third-generation cephalosporins remain the agents of choice for gonorrhea. However, in cases of apparent relapse, cultures for N gonorrhoeae followed by susceptibility testing are recommended to monitor the trend of emerging cephalosporin resistance.
Until 1962, meningococci were uniformly susceptible to sulfonamides, and these drugs were effective for both prophylaxis and therapy. Subsequently, sulfonamide-resistant meningococci spread widely, and the sulfonamides have now lost their usefulness against meningococcal infections. Penicillins remain effective for therapy, and until recently, rifampin was used for prophylaxis. However, rifampin-resistant meningococci have emerged (as high as 27% of isolates), which may cause invasive infections. Fluoroquinolones have largely replaced rifampin for prophylaxis.
In 1944, most staphylococci were susceptible to penicillin G, although a few resistant strains had been observed. After massive use of penicillin, 65–85% of staphylococci isolated from hospitals in 1948 were β-lactamase producers and thus resistant to penicillin G. The advent of β-lactamase–resistant penicillins (eg, nafcillin, methicillin, oxacillin) provided a temporary respite, but infections caused by methicillin-resistant S aureus (MRSA) are common. Presently, penicillin-resistant staphylococci include not only those acquired in hospitals but also 80–90% of those isolated in the community. These organisms also tend to be resistant to other drugs (eg, tetracyclines). Likewise, MRSA are common in both community-onset infections caused by circulating clones such as USA 300 and in hospital-acquired infections. Health care–associated infections may be caused by either the more susceptible community strains or the typical multidrug-resistant nosocomially acquired clones. Vancomycin has been the major drug used for treatment of MRSA infections, but recovery of isolates with intermediate resistance and the reports of several cases of high-level resistance to vancomycin have spurred the search for newer agents. Some of the newer agents with activity against MRSA include daptomycin; linezolid; quinupristin–dalfopristin; and a novel cephalosporin agent, ceftaroline.
S pneumoniae was uniformly susceptible to penicillin G until 1963, when relatively penicillin-resistant strains were found in New Guinea. Penicillin-resistant pneumococci subsequently were found in South Africa, Japan, Spain, and later worldwide. In the United States, approximately 10% of pneumococci are resistant to penicillin G (minimum inhibitory concentrations [MICs] >2 μg/mL), and approximately 18% are intermediate (MICs, 0.1–1 μg/mL). The penicillin resistance is attributable to altered PBPs. Penicillin resistance in pneumococci tends to be clonal. Pneumococci also are frequently resistant to trimethoprim–sulfamethoxazole, erythromycin, and tetracycline. Quinolone resistance is also beginning to emerge because of increased usage and is caused by mutations in DNA topoisomerase IV or GyrA or GyrB of DNA gyrase.
The enterococci have intrinsic resistance to multiple antimicrobials, including penicillin G and ampicillin with high MICs, cephalosporins with very high MICs, low-level resistance to aminoglycosides, and resistance to trimethoprim–sulfamethoxazole in vivo. The enterococci also have shown acquired resistance to almost all other antimicrobials as follows: altered PBPs and resistance to β-lactams; high-level resistance to aminoglycosides; and resistance to fluoroquinolones, macrolides, azalides, and tetracyclines. Some enterococci have acquired a plasmid that encodes for β-lactamase that renders them fully resistant to penicillin and ampicillin. Of greatest importance is the development of resistance to vancomycin, which has become common in Europe and North America, although there is geographic variation in the percentages of enterococci that are vancomycin resistant. Enterococcus faecium is the species that is most commonly vancomycin resistant. In outbreaks of infections caused by vancomycin-resistant enterococci (VRE), the isolates may be clonal or genetically diverse. Resistance to the streptogramins (quinupristin–dalfopristin) also occurs in enterococci. Increasing resistance to active drugs for VRE treatment such as linezolid is of major concern.
Most drug resistance in enteric bacteria is attributable to the widespread transmission of resistance plasmids among different genera. About half the strains of Shigella species in many parts of the world are now resistant to multiple drugs.
Salmonellae carried by animals have also developed resistance, particularly to drugs (especially tetracyclines) incorporated into animal feeds. The practice of incorporating drugs into animal feeds causes farm animals to grow more rapidly but is associated with an increase in drug-resistant enteric organisms in the fecal microbiota of farm workers. A concomitant rise in drug-resistant Salmonella infections in Britain led to a restriction on antibiotic supplements in animal feeds. Continued use of tetracycline supplements in animal feeds in the United States may contribute to the spread of resistance plasmids and drug-resistant salmonellae. In the late 1990s, a clone of Salmonella serotype Typhimurium phage type DT104 emerged and spread globally. This particular strain is resistant to ampicillin, chloramphenicol, streptomycin, sulfonamides, and tetracycline.
Plasmids carrying drug resistance genes occur in many gram-negative bacteria of the normal gut microbiota. The abundant use of antimicrobial drugs—particularly in hospitalized patients—leads to the suppression of drug-susceptible organisms in the gut microbiota and favors the persistence and growth of drug-resistant bacteria, including Enterobacter, Klebsiella, Proteus, Pseudomonas, and Serratia species and fungi. Such organisms present particularly difficult problems in granulocytopenic and immunocompromised patients. The closed environments of hospitals favor transmission of such resistant organisms through personnel and fomites as well as by direct contact.
Primary drug resistance in M tuberculosis occurs in about 10% of isolates and most commonly is to INH or streptomycin. Resistance to rifampin or ethambutol is less common. INH and rifampin are the primary drugs used in most standard treatment regimens; other first-line drugs are pyrazinamide and ethambutol. Resistance to INH and rifampin is considered multiple drug resistance. In the United States, multiple drug resistance of M tuberculosis (MDR-TB) has significantly decreased. Worldwide, the highest rates of MDR-TB have been reported from Eastern European countries, particularly among countries of the former Soviet Union. Poor compliance with drug treatment is a major factor in the development of drug resistance during therapy. Control of MDR-TB is a significant worldwide problem. More recently, emergence of extensively drug-resistant TB (XDR-TB) presents a significant challenge to global tuberculosis control. In addition to resistance to INH and rifampin, these organisms are also resistant to quinolones and injectable drugs such as aminoglycosides or capreomycin (second-line agents).
ANTIMICROBIAL ACTIVITY IN VITRO
Antimicrobial activity is measured in vitro to determine (1) the potency of an antibacterial agent in solution, (2) its concentration in body fluids or tissues, and (3) the susceptibility of a given microorganism to known concentrations of the drug.
Among the many factors that affect antimicrobial activity in vitro, the following must be considered because they significantly influence the results of tests.
Some drugs are more active at an acid pH (eg, nitrofurantoin); others are more active at an alkaline pH (eg, aminoglycosides, sulfonamides).
Sodium polyanetholsulfonate (in blood culture media) and other anionic detergents inhibit aminoglycosides. PABA in tissue extracts antagonizes sulfonamides. Serum proteins bind penicillins in varying degrees, ranging from 40% for methicillin to 98% for dicloxacillin. Addition of NaCl to the medium enhances the detection of methicillin resistance in S aureus.
At incubator temperature, several antimicrobial agents lose their activity. Penicillins are inactivated slowly, but aminoglycosides and ciprofloxacin are quite stable for long periods.
In general, the larger the bacterial inoculum, the lower the apparent “susceptibility” of the organism. Large bacterial populations are less promptly and completely inhibited than small ones. In addition, a resistant mutant is much more likely to emerge in large populations.
In many instances, microorganisms are not killed but only inhibited upon short exposure to antimicrobial agents. The longer incubation continues, the greater the chance for resistant mutants to emerge or for the least susceptible members of the antimicrobial population to begin multiplying as the drug deteriorates.
In general, actively and rapidly growing organisms are more susceptible to drug action than those in the resting phase. Metabolically inactive organisms that survive long exposure to a drug may have offspring that are fully susceptible to the same drug.
Determination of the susceptibility of a bacterial pathogen to antimicrobial drugs can be done by one of two principal methods: dilution or diffusion. It is important to use a standardized method that controls for all the factors that affect antimicrobial activity. In the United States, the tests are performed according to the methods of the Clinical and Laboratory Standards Institute (CLSI). These tests also are discussed in Chapter 47.
Using an appropriate standard test organism and a known sample of drug for comparison, these methods can be used to estimate either the potency of antibiotic in the sample or the susceptibility of the microorganism.
Graded amounts of antimicrobial substances are incorporated into liquid or solid bacteriologic media. Commonly, twofold (log2) dilutions of the antimicrobial agents are used. The media are subsequently inoculated with test bacteria and incubated. The end point is taken as that amount of antimicrobial substance required to inhibit the growth of—or to kill—the test bacteria. Agar dilution susceptibility tests are time consuming, and their use is limited to special circumstances. Broth dilution tests were cumbersome and little used when dilutions had to be made in test tubes; however, the advent of prepared broth dilution panels for many different drugs in manual or automated microdilution plates (eg, Vitek 2 instrument, bioMerieux, Inc.) has greatly enhanced and simplified the method. The advantage of microbroth dilution tests is that they permit a quantitative result to be reported, indicating the amount of a given drug necessary to inhibit (minimum inhibitory concentration, MIC) or kill (minimum bactericidal concentration, MBC) the microorganisms tested.
A widely used method in smaller laboratories is the disk diffusion test. A filter paper disk containing a measured quantity of a drug is placed on the surface of a solid medium that has been inoculated on the surface with the test organism. After incubation, the diameter of the clear zone of inhibition surrounding the disk is taken as a measure of the inhibitory power of the drug against the particular test organism. This method is subject to many physical and chemical factors in addition to the simple interaction of drug and organisms (eg, the nature of the medium and diffusibility, molecular size, and the stability of the drug). Nevertheless, standardization of conditions permits determination of the susceptibility of the organism.
Interpretation of the results of diffusion tests must be based on comparisons between dilution and diffusion methods. Such comparisons have led to the establishment of reference standards. Linear regression lines can express the relationship between log of minimum inhibitory concentration in dilution tests and diameter of inhibition zones in diffusion tests.
Use of a single disk for each antibiotic with careful standardization of the test conditions permits the report of susceptible or resistant for a microorganism by comparing the size of the inhibition zone with a standard of the same drug.
Inhibition around a disk containing a certain amount of antimicrobial drug does not imply susceptibility to that same concentration of drug per milliliter of medium, blood, or urine.
ANTIMICROBIAL ACTIVITY IN VIVO
Analysis of the activity of antimicrobial agents in vivo is much more complex than the circumstances in vitro. The activity involves not only the drug and organism but also a third factor, the host. Drug–pathogen and host–pathogen relationships are discussed in the following paragraphs. Host–drug relationships (absorption, excretion, distribution, metabolism, and toxicity) are dealt with mainly in pharmacology texts.
Several important interactions between drug and pathogen have been discussed in the preceding pages. The following are additional important in vivo factors.
In the host, varying environmental influences affect microorganisms located in different tissues and in different parts of the body—in contrast to the test tube or Petri dish, where the environment is constant for all members of a microbial population. Therefore, the response of the microbial population is much less uniform within the host than in the test tube.
In the body, the state of metabolic activity is diverse—undoubtedly, many organisms exist at a low level of biosynthetic activity and are thus relatively insusceptible to drug action. These “dormant” microorganisms often survive exposure to high concentrations of drugs and subsequently may produce a clinical relapse of the infection.
In the body, the antimicrobial agent is unequally distributed in tissues and fluids. Many drugs do not reach the central nervous system (CNS) effectively. The concentration in urine is often much greater than the concentration in blood or other tissue. The tissue response induced by the microorganism may protect it from the drug. Necrotic tissue or pus may adsorb the drug and thus prevent its contact with bacteria.
In the body, microorganisms often are located within tissue cells. Drugs enter tissue cells at different rates. Some (eg, tetracyclines) reach about the same concentration inside monocytes as in the extracellular fluid. With others (eg, gentamicin), the drug probably does not enter host cells at all. This is in contrast to the test tube, where microorganisms come into direct contact with the drug.
The biochemical environment of microorganisms in the body is very complex and results in significant interference with drug action. The drug may be bound by blood and tissue proteins or phospholipids; it may also react with nucleic acids in pus and may be physically adsorbed onto exudates, cells, and necrotic debris. In necrotic tissue, the pH may be highly acid and thus unfavorable for drug action (eg, aminoglycosides).
In the body, microorganisms are not exposed to a constant concentration of drug; in the test tube, they are.
The absorption of drugs from the intestinal tract (if taken by mouth) or from tissues (if injected) is irregular. There is also a continuous excretion as well as inactivation of the drug. Consequently, the levels of drug in body compartments fluctuate continually, and the microorganisms are exposed to varying concentrations of the antimicrobial agent.
The distribution of drugs varies greatly with different tissues. Some drugs penetrate certain tissues poorly (eg, CNS, prostate). Drug concentrations after systemic administration may therefore be inadequate for effective treatment. On surface wounds or mucous membranes such as the conjunctivae, local (topical) application of poorly absorbed drugs permits highly effective local concentrations without toxic side effects. Alternatively, some drugs applied topically on surface wounds are well absorbed. Drug concentrations in urine are often much higher than in blood.
It is critical to maintain an effective concentration of a drug where the infecting microorganisms proliferate. This concentration must be maintained for a sufficient length of time to eradicate the microorganisms. Because the drug is administered intermittently and is absorbed and excreted irregularly, the levels constantly fluctuate at the site of infection. To maintain sufficient drug concentrations for a sufficient time, the time–dose relationship must be considered. The larger each individual drug dose, the longer the permissible interval between doses. The smaller the individual dose, the shorter the interval that will ensure adequate drug levels.
The postantibiotic effect is the delayed regrowth of bacteria after exposure to antimicrobial agents. It is a property of most antimicrobials except that most β-lactams do not show the postantibiotic effect with gram-negative bacilli. The carbapenems do have a postantibiotic effect with the gram-negative bacilli. Aminoglycosides and fluoroquinolones have prolonged (up to several hours) in vitro postantibiotic effects against gram-negative bacilli.
Host–pathogen relationships may be altered by antimicrobial drugs in several ways.
The inflammatory response of the tissue to infections may be altered if the drug suppresses the multiplication of microorganisms but does not eliminate them from the body. An acute process may in this way be transformed into a chronic one. Conversely, the suppression of inflammatory reactions in tissues by impairment of cell-mediated immunity in recipients of tissue transplants or antineoplastic therapy or by immunocompromise as a result of disease (eg, AIDS) causes enhanced susceptibility to infection and impaired responsiveness to antimicrobial drugs.
If an infection is modified by an antimicrobial drug, the immune response of the host may also be altered. One example illustrates this phenomenon: Pharyngeal infection with β-hemolytic group A streptococci is followed frequently by the development of antistreptococcal antibodies, and if there is a hyperimmune response, the infection may be followed by rheumatic fever. If the infective process can be interrupted early and completely with antimicrobial drugs, the development of an immune response and of rheumatic fever can be prevented (presumably by rapid elimination of the antigen). Drugs and dosages that rapidly eradicate the infecting streptococci (eg, penicillin) are more effective in preventing rheumatic fever than those that merely suppress the microorganisms temporarily (eg, tetracycline).
Antimicrobial drugs affect not only the microorganisms causing disease but also susceptible members of the normal microbiota. An imbalance is thus created that in itself may lead to disease. A few examples are of interest.
In hospitalized patients who receive antimicrobials, the normal microbiota is suppressed. This creates a partial void that is filled by the organisms most prevalent in the environment, particularly drug-resistant gram-negative aerobic bacteria (eg, pseudomonads, staphylococci). Such superinfecting organisms subsequently may produce serious drug-resistant infections.
In women taking antibiotics by mouth, the normal vaginal microbiota may be suppressed, permitting marked overgrowth of Candida. This leads to unpleasant local inflammation (vulvovaginitis) and itching that are difficult to control.
In the presence of urinary tract obstruction, the tendency to bladder infection is great. When such urinary tract infection due to a susceptible microorganism (eg, E coli
Stay updated, free articles. Join our Telegram channel
Full access? Get Clinical Tree
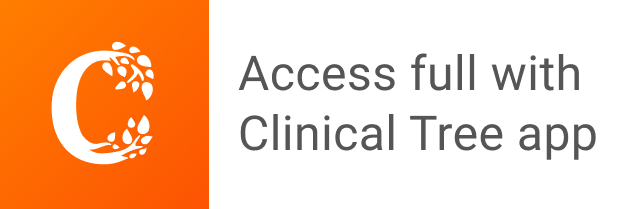