Chapter 2
- 1.
The mRNA sequence is 5′-CAG AAG AAA AUU AAC AUG UAA-3′ (remember that transcription moves along the 3′–5′ DNA strand, allowing the mRNA to be synthesized in the 5′ to 3′ direction). This mRNA sequence is translated in the 5′ to 3′ direction to yield the following amino acid sequence: Gln-Lys-Lys-Ile-Asn-Met-STOP.
- 2.
The genome is the sum total of our genetic material. It is composed of 23 pairs of nuclear chromosomes and the mitochondrial chromosome. Each chromosome contains a number of genes, the basic unit of heredity. Genes are composed of one or more exons; exons alternate with introns. Exons encode mRNA codons, which consist of three nucleotides each. It is important to remember that DNA coiling patterns also produce a hierarchy: Chromosomes are composed of 100-kb chromatin loops, which are in turn composed of solenoids. Each solenoid contains approximately six nucleosomes. Each nucleosome contains about 150 DNA base pairs and may or may not include coding material.
- 3.
Approximately 55% of human DNA consists of repetitive sequences, whose function is largely unknown. Single-copy DNA includes protein-coding genes, but it consists mostly of extragenic sequences and introns that do not encode proteins. Because individual cells have specialized functions, most make only a limited number of protein products. Thus only a small percentage of the cell’s coding DNA is transcriptionally active at any given time. This activation is controlled by elements such as transcription factors, enhancers, and promoters.
- 4.
Mitosis is the cell division process whereby one diploid cell produces two diploid daughter cells. In meiosis, a diploid cell produces haploid cells (gametes). Meiosis produces haploid cells because the centromeres are not duplicated in meiosis I and because there is no replication of DNA in the interphase stage between meiosis I and meiosis II. Another difference between mitosis and meiosis is that the homologous chromosomes form pairs and exchange material (crossing over) during meiosis I. Homologs do not pair during mitosis, and mitotic crossing over is very rare.
- 5.
Each mitotic division doubles the number of cells in the developing embryo. Thus the embryo proceeds from 1 to 2, to 4 to 8 cells, and so on. After n cell divisions, there are 2 n cells. For example, after 10 divisions, there are 2 10 , or 1024, cells. We want a value of n that satisfies the simple relationship 2 n = 10 13 . One way to find our answer is simply to plug in values of n until we get 10 13 . A more elegant approach is to take the common logarithms of both sides of this equation, yielding n log(2) = 13log(10). Because the common logarithm of 10 is 1, we obtain the relationship n = 13/log(2). Thus n = 43.1. This result, approximately 43 cell divisions, is only an average value. Some cell lineages divide more times than others, and many cells are replaced as they die.
- 6.
A total of 400 mature sperm and 100 mature egg cells will be produced. Each primary spermatocyte produces four mature sperm cells, and each primary oocyte produces only one mature egg cell (the other products of meiosis are polar bodies, which degenerate).
Chapter 3
- 1.
Mutation 1 is a nonsense mutation in the fourth codon, which produces premature termination of translation. Mutation 2 is a frameshift mutation in the third codon, and mutation 3 is a missense mutation in the second codon.
- 2.
Transcription mutations generally lower the production of a gene product, but often they do not eliminate it completely. Transcription mutations in the β-globin gene usually produce β + -thalassemia, a condition in which there is some production of β-globin chains. β + -thalassemia tends to be less severe than β 0 -thalassemia. Missense mutations alter only a single amino acid in a polypeptide chain, and when they occur in the β-globin chain, they can produce β + -thalassemia. (However, keep in mind that sickle cell disease, which is relatively severe, is also caused by a missense mutation.) In contrast, frameshift mutations alter many or all codons downstream from the site of the mutation, so a large number of amino acids may be changed. Frameshifts can also produce a stop codon. Nonsense mutations produce truncated polypeptides, which are often useless (especially if the nonsense mutation occurs near the 5′ end of the gene, eliminating most of the polypeptide chain). Donor and acceptor mutations can delete whole exons or large portions of them. This deletion can substantially alter the amino acid composition of the polypeptide. Nonsense, frameshift, and donor or acceptor mutations all tend to produce the more-severe β 0 -thalassemia, in which no β-globin chains are present.
- 3.
In thalassemia conditions, one of the chains, α- or β-globin, is reduced in quantity. Most of the harmful consequences are caused by the relative excess of the chain that is produced in normal quantity. If both chains are reduced in quantity, there may be a rough balance between the two, resulting in less accumulation of excess chains.
- 4.
SNPs are single nucleotide polymorphisms, typically detected by microarrays or DNA sequencing. STRs consist of variations in the number of tandemly repeated DNA sequences (usually dinucleotides, trinucleotides, or tetranucleotides) at a specific location on a chromosome. They are detected with the use of the polymerase chain reaction (PCR) or by whole-genome sequencing. Because the number of tandem repeats can vary considerably, STRs can have many different alleles in populations. The accompanying figure represents an STR. This is indicated by the fact that there are multiple alleles (distinguishing it from a SNP) and by the fact that the various alleles differ in size by only 4 bp (recall from Chapter 2 that the tandem repeat units in minisatellite regions are generally more than 10 bp long). Indels are single (rather than multiple tandem) insertions or deletions of DNA sequence less than 50 bp. CNVs (copy number variants) are larger structural variants (500–1000 bp or larger) that can exist in multiple copies varying from zero to more than a dozen.
- 5.
Because the disease mutation destroys a recognition site, those who have the disease allele have a longer restriction fragment. This fragment migrates more slowly on the gel and is seen higher on the autoradiogram. Individual A has only the longer fragment and thus has two copies of the disease mutation. This individual has α 1 -antitrypsin deficiency. Individual B has only the short fragment and is genetically and physically unaffected. Individual C has both fragments and is thus a clinically unaffected heterozygote.
- 6.
In this sample of 100 individuals, there are 88 × 2 HbA alleles in the HbA homozygotes and 10 HbA alleles in the heterozygotes. There are thus 186 HbA alleles in the population. The frequency of HbA, p, is 186/200 = 0.93, and the frequency of HbS, q, is 1 − 0.93 = 0.07. The genotype frequencies in the population are 88/100 = 0.88, 10/100 = 0.10, and 2/100 = 0.02 for the HbA/HbA, HbA/HbS, and HbS/HbS genotypes, respectively. Assuming Hardy–Weinberg proportions, the expected genotype frequencies are given by p 2 , 2 pq, and q 2 , respectively. This yields expected genotype frequencies of (0.93) 2 = 0.865, 2 × 0.93 × 0.07 = 0.130, and (0.07) 2 = 0.005, respectively. In this population, the observed and expected genotype frequencies are fairly similar to each other.
- 7.
For an autosomal recessive disease, the prevalence (1/10,000) equals the recessive genotype frequency, q 2 . Thus the PKU gene frequency, q, is given by √ q 2 = √1/10,000 = 1/100 = 0.01. The carrier frequency is given by 2 pq, which is approximately 2 q, or 0.02 (i.e., 1/50).
Chapter 4
- 1.
Because this is an autosomal dominant disorder, and because affected homozygotes die early in life, the man is a heterozygote and has a 50% chance of passing the disease-causing allele to each of his offspring. The probability that all four will be affected is given by the product of each probability: (1/2) 4 = 1/16. The probability that none will be affected (1/16) is obtained in exactly the same way.
- 2.
The probability that the offspring will inherit the retinoblastoma susceptibility allele is 0.50, because familial retinoblastoma is an autosomal dominant disease. However, we must also consider the penetrance of the disorder. The probability of both inheriting the disease-causing allele (0.50) and expressing the disease phenotype (0.90) is given by multiplying the two probabilities together: 0.90 × 0.50 = 0.45.
- 3.
Because the woman’s sister had Tay–Sachs disease, both parents must be heterozygous carriers. This means that at birth, one-fourth of their offspring will be affected, one-half will be carriers, and one-fourth will be genetically normal. Note, however, that the woman in question is 30 years old. She cannot possibly be an affected homozygote because affected individuals die by age 6 years. There are thus three equally likely possibilities: (1) the disease allele was inherited from the mother and a normal allele was inherited from the father; (2) the disease allele was inherited from the father and a normal allele was inherited from the mother; (3) normal alleles were inherited from both parents. Because two of these three possibilities lead to the carrier state, the woman’s probability of being a heterozygous carrier is 2/3.
- 4.
Because the mother has neurofibromatosis and can be assumed to be a heterozygote (homozygotes for this condition have not been reported), the probability that her daughter (the man’s sister) inherits the disease-causing allele is 1/2. The probability that the sister transmits the disease-causing allele to her daughter is again 1/2, so the probability that both events occur is 1/2 × 1/2 = 1/4. If we knew that the man’s sister was affected, then the probability that his sister’s daughter is affected is simply 1/2.
- 5.
The probability that the woman’s disease-causing allele is transmitted to her offspring is 1/2, and the probability that this offspring in turn transmits the disease-causing allele to his or her offspring (i.e., the grandchild) is again 1/2. Thus the probability that one grandchild has inherited the allele is 1/2 × 1/2, or 1/4. Similarly, the probability that the other grandchild has inherited the allele is 1/4. The probability that both grandchildren have inherited the allele is 1/4 × 1/4 = 1/16. If the grandmother has PKU, she must be homozygous for the disease-causing allele. Thus both of her children must be heterozygous carriers (probability = 1). The probability that one of these individuals transmits the disease-causing allele to their offspring is 1/2. The probability that they both transmit the disease-causing allele to their offspring (i.e., that both grandchildren are heterozygous carriers) is 1/2 × 1/2 = 1/4.
- 6.
The coefficient of relationship is (1/2) 6 , or 1/64. This gives the probability that the second member of the couple carries the PKU allele. The probability that two carriers will produce an affected offspring is 1/4. The overall probability that this couple will produce a baby affected with PKU is given by multiplying the probability that the mate also carries the allele (1/64) by the probability that the couple both transmit the allele to their offspring (1/4): 1/64 × 1/4 = 1/256. This demonstrates that the probability of producing an affected offspring in this consanguineous mating is actually quite small.
- 7.
The frequency of the heterozygote genotype in the general population is given by 2 pq, according to the Hardy–Weinberg law. Thus the frequency of the first genotype in the general population is predicted to be 2 × 0.05 × 0.10 = 0.01. Similarly, for the second system, the fre quency of heterozygotes in the general population is 2 × 0.07 × 0.02 = 0.0028. The perpetrator was a homozygote for the third system; the frequency of the homozygote genotype in the general population is given by p 2 , or 0.08 2 = 0.0064. If we can assume independence of the three STR loci in the general population, we can then multiply the frequencies of the three genotypes together to get the probability that a randomly chosen individual in the general population would have the same genotypes as the perpetrator. We thus multiply 0.01 × 0.0028 × 0.0064 to obtain a probability of 0.000000179, or 1/5, 580,357. This probability can be made smaller by typing additional loci.
- 8.
As in question 7, we assume independence of the four STR loci. In this case, we multiply the four allele frequencies together to get the probability: 0.05 × 0.01 × 0.01 × 0.02 = 0.0000001 = 1/10,000,000. Notice a key difference between question 8 and question 7. In the paternity case, the father has contributed only half of the baby’s genotype at each locus, the other half being contributed by the mother. Thus we examine only a single allele for each locus. In contrast, the rapist has contributed both alleles of each genotype to the evidentiary sample, so we need to know the frequency of each genotype in the general population. We use the Hardy–Weinberg law to estimate the population frequency of each genotype, based on the known allele frequencies.
Chapter 5
- 1.
There are four Barr bodies, always one less than the number of X chromosomes.
- 2.
This is most likely a result of X inactivation. The heterozygotes with muscle weakness are the ones with relatively large proportions of active X chromosomes containing the mutant allele.
- 3.
The disease frequency in males is q, and in females it is q 2 . The male-to-female ratio is thus q / q 2 or 1/ q. Thus as q decreases, the male-to-female ratio increases.
- 4.
Because the male’s grandfather is affected with the disorder, his mother must be a carrier. His father is phenotypically normal and therefore does not have the disease allele. Thus the male in question has a 50% risk of developing hemophilia A. His sister’s risk of being a heterozygous carrier is also 50%. Her risk of being affected with the disorder is close to zero (barring a new mutation on the X chromosome transmitted by her father).
- 5.
Male-to-male transmission can be observed in autosomal dominant inheritance, but it is not observed in X-linked dominant inheritance. Thus males affected with X-linked dominant disorders must always have affected mothers, unless a new mutation has occurred. Males and females are affected in approximately equal proportions in autosomal dominant inheritance, but there are twice as many affected females as males in X-linked dominant inheritance (unless the disorder is lethal prenatally in males, in which case only affected females are seen). In X-linked dominant inheritance, all of the sons of an affected male are normal, and all of the daughters are affected. In X-linked dominant diseases, heterozygous females tend to be more mildly affected than are hemizygous males. In autosomal dominant inheritance, there is usually no difference in severity of expression between male and female heterozygotes.
- 6.
In mitochondrial inheritance, the disease can be inherited only from an affected mother. In contrast to all other types of inheritance, no descendants of affected fathers can be affected. Note that males with an X-linked recessive disease who mate with normal females cannot transmit the disease to their offspring, but their grandsons can be affected with the disease.
- 7.
Because Becker muscular dystrophy is relatively rare, it is reasonable to assume that the woman is a normal homozygote. Thus she can transmit only normal X chromosomes to her offspring. Her male offspring, having received a Y chromosome from the father and a normal X from the mother, will all be unaffected. All female offspring of this mating will receive a mutated X chromosome from the father, and all will be heterozygous carriers of the disorder.
- 8.
If the man is phenotypically normal, his X chromosome cannot carry a Duchenne muscular dystrophy mutation. The female will transmit her mutation-carrying chromosome to half of her offspring, on average. Thus half of the sons will be affected with the disorder, and half of the daughters will be heterozygous carriers.
- 9.
The boys’ mother must be a heterozygous carrier for a factor VIII mutation. Thus one of the mother’s parents must also have carried the mutation. Consequently, the probability that the mother’s sister is a carrier is 1/2.
- 10.
This is explained by genomic imprinting. For normal development, differential expression of genes inherited from the father and mother is necessary. If the expression pattern from only one parent is inherited, the embryo cannot develop normally and dies. The experiment works in amphibians because genomic imprinting does not occur in these animals.
- 11.
Autosomal dominant. Male-male transmission is seen (excluding X-linked inheritance), males and females are affected, and the disease phenotype is seen in each generation. The unaffected obligate carrier father in generation 3 is an example of incomplete penetrance. Autosomal recessive inheritance is ruled out because several heterozygous carriers would have to have “married into” this pedigree. Because the gene frequency is low, this is highly unlikely.
- 12.
X-linked recessive. Only males are affected, there is no male-male transmission, and a skipped generation is seen: affected male—unaffected female—affected male.
- 13.
Autosomal recessive. Two generations show no affected individuals, males and females are affected, and consanguinity (double bar) is present, resulting in the mating of two heterozygous carriers in generation 3.
- 14.
X-linked dominant. Phenotype is seen in every generation, ratio of affected females to affected males is 2:1, and there is no male-male transmission.
- 15.
X-linked recessive. Only males are affected, there is no male-male transmission, and a skipped generation is seen: affected male—unaffected female—affected male.
- 16.
Y-linked. Only males are affected, and transmission is exclusively male-male.
- 17.
Mitochondrial (with complete penetrance). Both sexes are affected, but only females transmit the phenotype to offspring.
- 18.
Autosomal dominant. Males and females are affected in roughly equal proportion, male-male transmission is seen, and the phenotype is seen in every generation.
Chapter 6
- 1.
Euploid cells have a multiple of 23 chromosomes. Haploid ( n = 23), diploid ( n = 46), and polyploid (triploid and tetraploid) cells are all euploid. Aneuploid cells do not have a multiple of 23 chromosomes, and include trisomies (47 chromosomes in a somatic cell) and monosomies (45 chromosomes in a somatic cell).
- 2.
Conventional FISH analysis involves the hybridization of a fluorescently labeled probe to denatured metaphase chromosomes and is most useful in detecting aneuploidy, deletions, and chromosome rearrangements. FISH can also be extended to use multiple differently colored probes, allowing the detection of several different aneuploidies simultaneously. Spectral karyotyping is an extension of FISH in which each chromosome can be visualized as a different color. This enables accurate and easy characterization of aneuploidy, loss or duplication of chromosome material, and (especially) chromosome rearrangements such as translocations. CGH is particularly useful in detecting the gain or loss of chromosome material, but it cannot detect balanced rearrangements of chromosomes, such as reciprocal translocations. This is because, despite the rearrangement, the test sample and the reference sample each have the same amount of DNA from each chromosome region. CMA has higher resolution than CGH and can detect uniparental disomy and loss of heterozygosity, as well as deletions and duplications.
- 3.
A normal egg can be fertilized by two sperm cells (dispermy, the most common cause of triploidy). An egg and polar body can fuse, creating a diploid egg, which is then fertilized by a normal sperm cell. Diploid sperm or egg cells can be created by meiotic failure; subsequent union with a haploid gamete would produce a triploid zygote.
- 4.
The difference in incidence of various chromosome abnormalities reflects the fact that embryos and fetuses with chromosome abnormalities are spontaneously lost during pregnancy. The rate and timing of loss vary among different types of chromosome abnormalities.
- 5.
A karyotype establishes whether the condition is the result of a true trisomy or a translocation. If the trisomy is a translocation, the recurrence risk in future pregnancies is greatly elevated. A karyotype also helps to establish whether the patient is a mosaic. This can help to predict and explain the severity of expression of the disorder.
- 6.
The risk, from lowest risk to highest, is (1) 25-year-old woman with one previous Down syndrome child (approximately 1%); (2) 25-year-old male carrier of a 21/14 translocation (1–2%); (3) 45-year-old woman with no family history (approximately 3%); (4) 25-year-old female carrier of a 21/14 translocation (10–15%).
- 7.
Nondisjunction of the X chromosome can occur in both meiosis I and meiosis II. If these two nondisjunctions occur in the same cell, an ovum with four X chromosomes can be produced. If this is fertilized by an X-bearing sperm cell, the zygote will have the 49,XXXXX karyotype.
- 8.
D. Cytogenomic array. The clinical presentation suggests trisomy 13, and a cytogenomic array would reveal this, as well as other duplications or deletions (making it superior to a karyotype). A brain MRI is not needed, and exome sequencing (with interpretation of the results) would be more expensive than the cytogenomic array.
- 9.
The meiotic error must have occurred in the father, because his X chromosome carries the gene for hemophilia A. Because the daughter has normal factor VIII activity, she must have inherited her single X chromosome from her mother.
- 10.
A loss of genetic material usually produces more severe consequences than does a gain of material, so one would expect the patient with the deletion—46,XY,del(8p)—to be more severely affected than the patient with the duplication.
- 11.
A translocation can place a proto-oncogene near a sequence that activates it, producing a cancer-causing oncogene. It can also interrupt a tumor-suppressor gene (see Chapter 11 ), inactivating it. Because these genes encode tumor-suppressing factors, their inactivation can also lead to cancer.
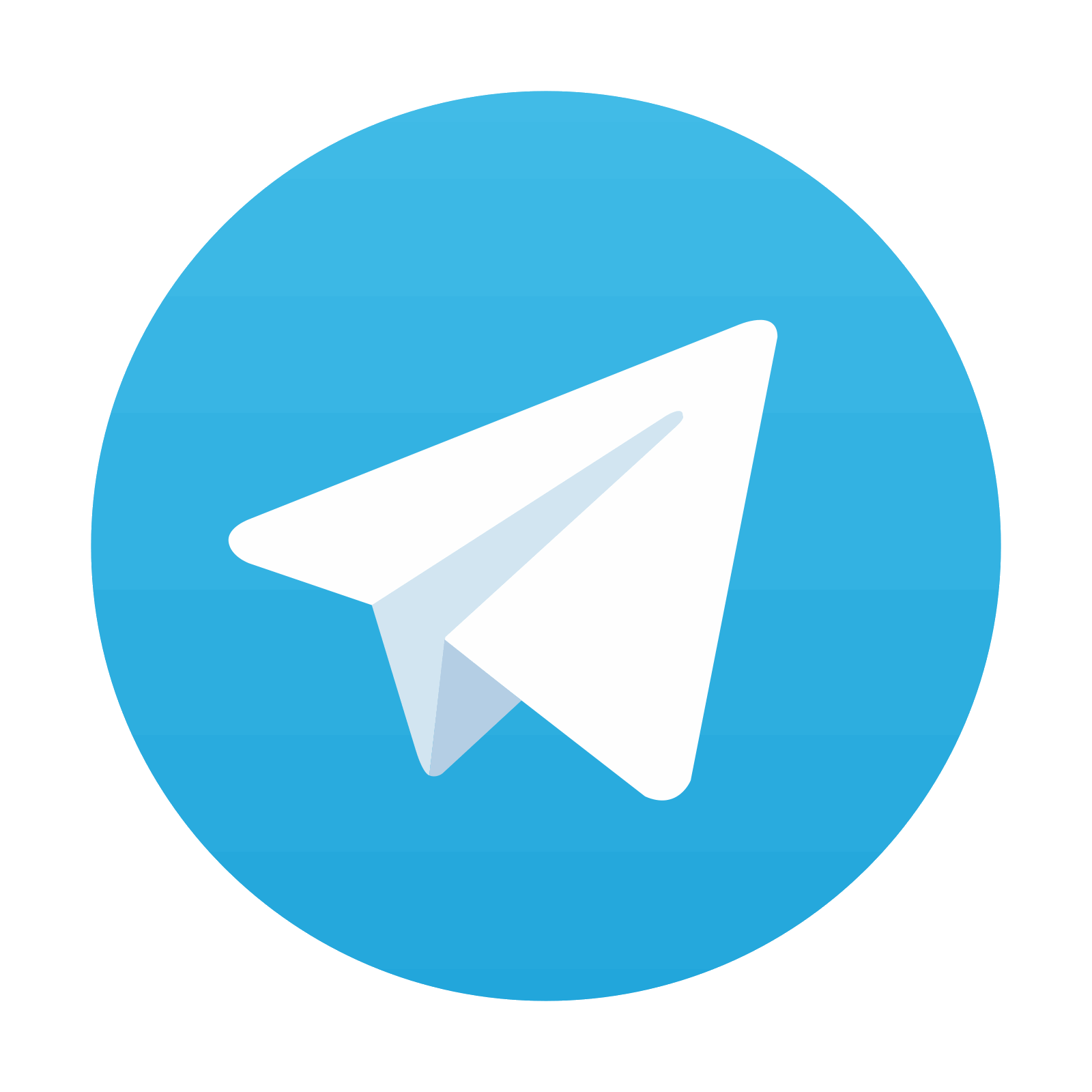
Stay updated, free articles. Join our Telegram channel
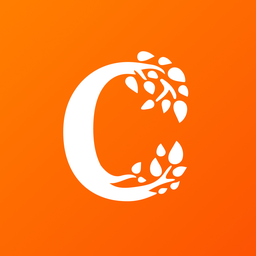
Full access? Get Clinical Tree
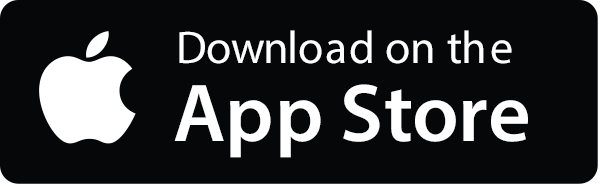
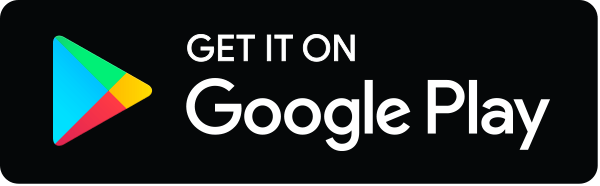